Sweetening of Natural Gas Through Hollow Silica Nanoparticles Embedded Hydroxyethyl Cellulose Membrane
Mallikarjunagouda B. Patil1*
, Amshumali M. K2
1Department of Chemistry, Basaveshwar Science College, Bagalkot, Karnataka, India
2Department of Chemistry, Vijayanagara Sri Krishnadevaraya University, Ballar, Karnataka, India.
Corresponding Author E-mail: mallupatil04@gmail.com
DOI : http://dx.doi.org/10.13005/msri/150308
Article Publishing History
Article Received on : 11-Oct-2018
Article Accepted on : 07-Dec-2018
Article Published : 11 Dec 2018
Plagiarism Check: Yes
Reviewed by: Mochamad Asrofi
Second Review by: Pranay Agarwal
Final Approval by: Alokmay Datta
Article Metrics
ABSTRACT:
Membrane gas separation technique is a promising technique for separation of gases. Nanoparticles (NPs) of hollow spherical silica were synthesized by the hydrolysis and condensation of tetramethylorthosilicate (TMOS). The nanocomposite membranes were prepared by dispersing hollow silica in hydroxyethylcellulose (HEC) polymeric solution and were cast as membranes by solution casting-solvent evaporation procedure. The % loading of NP’s in membrane varied from 0.5 to 2 Wt. %. The effects of experimental parameters such as gas permeability and selectivity at constant pressure were measured for gas transport properties for the prepared membranes. Under constant operating conditions of feed pressure (4.0 MPa) and membrane thickness (50 μm), the CO2 permeability was determined to be in the range 1790-3620 Barrer for nanocomposite membrane from 0.5 to 2 Wt. %, while selectivity declined from 3.7 to 3.1 due to plasticization. This made us to come to the conclusion that the membrane has a double mode of sorption effect. The membranes were characterized with FTIR to confirm the formation of compound and with SEM to study the distribution of nanoparticles in the membrane matrix.
KEYWORDS:
Polymeric membrane; Single gas permeation; CO2/CH4 separation; Selectivity
Copy the following to cite this article:
Patil M. B, Amshumali M. K. Sweetening of Natural Gas Through Hollow Silica Nanoparticles Embedded Hydroxyethyl Cellulose Membrane. Mat.Sci.Res.India;15(3).
|
Copy the following to cite this URL:
Patil M. B, Amshumali M. K. Sweetening of Natural Gas Through Hollow Silica Nanoparticles Embedded Hydroxyethyl Cellulose Membrane. Mat.Sci.Res.India;15(3). Available from: http://www.materialsciencejournal.org/?p=12159
|
Introduction
Separation and purification processes comprise an essential part of chemical industry for the isolation of product and revival of reactants as well as solvents. Membrane based separation process is a very recently developed technique and is a subject of great interest. It attracted a greater interest in Industry because of its minimum capital interest, lower operating cost and operational safety. Separation of binary gas mixture is an essential unit operation many of the industries to recover costly gases and to isolate pollutant gases. At present such operations are at present carried out by conventional technologies such as adsorption on solid surface, solvent scrubbing or steam stripping. In recent days, membranes have gained importance in this field for gaseous mixture separation and isolation of pollutant gases. In membrane-based separation technology, the development of an efficient membrane is the crucial focus of research. The main goal of developing membrane is to enhance the permeability and permselectivity.1-3
Several polymeric membranes were employed in sweetening of natural gas. From the very beginning, for sweetening of natural gas, cellulose acetate/triacetate and polyimide have been employed because of the high-performance fixed-site-carrier (FSC) membranes which have ability to show a great enhancement for CO2/CH4 separation.4 In the membrane gas separation process, high-pressure operation is a very critical challenge in case of natural gas purification with membrane separation technique. The plasticization effect observed in the most of the membranes is due to the glassy polymer nature and the high pressure and concentration gradients on the surface of the film. This absorbs a large amount of CO2 at elevated pressure,5-6 by yielding significant decline in the permeability of CO2 and results in decrease of selectivity of CO2 in CO2/ CH4 mixture. To overcome this problem, several approaches are made by scientists and have been reported earlier, the successful methods being membrane cross-linking7 and tailoring of high mechanical strength membranes that can be achieved by mixed matrix membrane (MMM), i.e. inorganic filler addition to the polymer matrix. As previously reported, Adams et al., prepared a poly (vinyl acetate) (PVAc) with 50 % (vol.) zeolite 4A MMM and used in CO2 separation from CO2/ CH4 mixture.8 In the findings, the MMM’s can come up to Robeson CO2/CH4 upper bound while permeability of CO2 remained unchanged with 63 % enhancement in selectivity in comparison with its pristine PVAc.
Several categories of polymeric membranes are becoming strong candidates in commercializing the membrane and have proven to be better compared to conventional cryogenic and absorption processes that are employed in gas separations,9 hydrogen recovery10as well as nitrogen making.11
Cellulose acetate membranes were used as a standard membrane due to their existing utilization in the processing of natural gas and their great resistance towards benzene, toluene, and xylene (BTX) streams.12 Several approaches were made to synthesize and to study different dimensions of membranes for separation of O2/N2 in air, CO2/CH4 from natural gas or biogas, CO2/N2 from flue gas, hydrogen recovery in the manufacture of ammonia and in the separation of olefin–paraffin in the refinery industry. Polymeric membranes are cast with several polymers such as matrimid, polysulfone, polyimides, polycarbonates, polyether imides, polyamides, cellulose acetate using various techniques.3, 13
A polysaccharide derivative of hydroxyethyl cellulose (HEC) has good water-retaining, emulsifying, stabilizing and bubble-forming properties. It is employed in formulations of topical drug to facilitate the drug delivery with hydrophobic character. The role of HEC has continued in polymer networks development and in block copolymers. It is successfully used in separation technology like capillary electrophoresis, in biofilms and as a coating agent.
In the membrane based separation technology, the development of a high performance membrane is the key role. In continuation of previous researcher’s effort, the present research work focuses on the improvement of the gas permeability and better permeability and selectivity of CO2.
Materials and Methods
Chemicals
The base polymer hydroxyl ethyl cellulose was purchased from Dow Chemicals and the solvent ethanol (99.9%) from Changshu Hongsheng Fine Chemical Co. Ltd, China. Tetramethyl orthosilicate was procured from E. Merck USA. HCl was purchased from S. D. Fine Chemicals, India. All the chemicals are used as such without further purification.
Synthesis of SiO2 Nanoparticles
The freshly prepared precursor of sol-gel silica was prepared by 1.0 M Si(OH)4 solution. The precursor silica is prepared by slow addition of tetramethyl orthosilicate to a 1 mM HCl solution and mixed well to get uniform solution. To synthesize hollow silica NPs, 50 μL of polystyrene beads suspension (2.64% by weight) 200 μL of 0.1% poly-L-lysine water solution were added to the amount of 1.0 mL maintaining pH 7.4 phosphate buffer (1X) followed by adding 25 μL of the Si(OH)4 solution and the mixture was stirred 15 min. Once the white solution is prepared, the solution is centrifuged using Remi R-12M centrifuge. The NPs were collected by centrifugation and washed by water several times to remove the unreacted substances. Polymer cores were removed by calcining particles for 18 h at 500 °C.
Preparation of self supportive films
The techniques adapted to cast a free standing self supportive polymeric membrane were solution casting and solvent evaporation method. The homogeneously mixed polymeric slurry of known amount i.e. 6 wt. % in ethanol solvent6 wt. % was prepared by stirring for 12 hrs to ensure that whole polymer is in a solution form. The prepared HEC polymeric solution was cast as membrane by the help of elicometer on the surface of a clean glass plate.
Preparation of Hydroxyethyl Cellulose-hollow Silica Membrane
The synthesized nanoparticles were washed thoroughly by water twice, topped up with the chloroform for the calculated amount using the balancing centrifuge technique. They were finally washed with the chloroform and collected in a vial. Then a known amount of highly viscous hydroxyl ethyl cellulose polymeric solution was slowly added into the vial till the determined weight is obtained, and then stirred well for 6 hrs to homogenize. The resultant mixture was cast on to a clean glass plate. Once the solution was dried, the dried composite polymeric membrane was slowly peeled off from the glass plate carefully and stored in a proper folder. The preparation of HEC and hollow silica membrane is illustrated in the Figure 1.
Figure 1: Schematic representation of nanoparticles embedded Nanocomposite HEC membrane preparation.
Fourier Transform Infrared Spectroscopy (FT-IR)
Small amount of the sample was mixed well with a portion of KBr and ground with the help of mortar and the obtained fine powder was made in to a thin pellet. The pellet was kept in a holder and scanned by FT-IR Shimadzu IR-Affinity-1S over the range 400 cm-1 to 4000 cm-1 (Figure 2).
Figure 2: FTIR tracings of (A) porous silica particles, (B) HEC polymer and (C) porous silica incorporated HEC membrane.
Scanning Electron Microscopy (SEM)
The surface morphology of the prepared plain and nano composite membranes were studied using the scanning electron microscopy to study of the membranes. SEM micrographs of the membranes were taken to see the distribution pattern and physical morphology of the hollow silica particles in the HEC matrix. The images of the membranes were taken at 12 keV as such without further conditioning. Scanning was done using a JEOL model JSM-840A (Japan) SEM. Selected SEM images are shown in Figure 3.
Figure 3: SEM Images of (a) Plain and (b) 1 wt. % Silica loaded membrane.
Permeability Measurements
The pure single gas permeabilities of N2 and CO2 were measured using a gas permeameter. The permeameter consists of a stainless steel permeation cell which separates upstream (feed side) and downstream (permeate side). A pressure transducer is connected to the downstream side to measure the pressure change with time in this side. The total effective membrane area exposed to the gas at one side is 13.302 cm2.14
The constant volume variable pressure model was used to measure the permeation of the pure gas. The gas pressure rise with respect to time was plotted from the rough data. The permeability of the gas is computed by using the following standard formula:

Where:
– P is the permeability of the gas through the membrane (barrer), (1 Barrer = 10-10 cm3 (STP) cm cm-2 s-1 cmHg-1).
– V is the permeate volume (cm3).
– l is the thickness of the membrane layer (cm).
– A is the effective area of the membrane (cm2).
The ideal selectivity αA/B of gas pair A and B was calculated. It is defined as the ratio of their permeabilities:
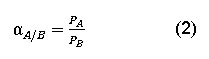
Results and Discussion
FTIR Studies
The spectrum of the synthesized silica particles is displayed in Figure 2(A) which confirms the presence of porous silica particles as reported elsewhere.15,16 The Si-O rocking vibration and Si-O bond stretching of the Si-OH moiety are observed around 460 cm-1 and 967 cm-1 respectively. A broad (hydrogen bonded) hydroxyl stretching (O-H) band for both silanol Si-O-H and the water hydroxyls are confirmed at 3500 cm-1. In the range of 1640 cm-1 a peak was observed which confirms the H-OH water twisting band. The peak at 1100 cm-1 confirms the presence of internal Si-O-Si asymmetric stretching vibration of SiO4 and the symmetric band is at 800 cm-1. The silicate network is formed of Si-O-Si and plenty of silanol Si-OH groups (both internal and external ones) of different types as described elsewhere.15, 17
FTIR spectroscopic investigations gave diverse absorption peaks that are characteristic of the hydroxyl ethyl cellulose as shown in figure 2(B). A broad band in the range of 3446 cm-1, characteristic for the OH-stretching vibration, provides information about the hydrogen bond formation. The band at 2900 cm-1 corresponds to the C–H stretching vibration. In addition, the absorption band at 1430 cm-1 confirms the symmetric CH2 bending vibration, the absorption band around 1000 cm-1 represents the C–O–C stretching at β-(1 -4)-glycosidic linkages of the cellulose, the absorption band at 1642 cm-1 corresponds to the absorbed H2O, and the absorption band at 1059 cm-1 represents the C–O stretch.
In the spectrum of 2 % silica nano particles embedded membranes of HEC displayed in Figure 2(C), apart from the prominent peaks of HEC, the extra peaks in the region 1640 cm-1 corresponding to the H-OH water twisting band of porous silica were observed and at 460 cm-1 region another peak is observed which represents the Si-OH bond. This confirms in the presence of porous silica nanoparticles in the prepared HEC matrix.
SEM Studies
The surface morphology of pristine and modified polymeric membranes was studied using scanning electron microscopy (SEM). The SEM images of plain and porous silica loaded HEC membranes are displayed in Figure 3. The images of the surface are taken at 20000× magnification. By the SEM image it is clear that the porous silica nanoparticles were distributed across the membrane surface but in an agglomerated and distributed non-uniform manner.
Gas Permeability Studies
Gas Permeability
The prepared membranes were assessed for the gas permeability measurement. Pure gas permeability was measured for H2, N2, CH4, and CO2 with the prepared hydroxyethyl cellulose (HEC) – hollow silica NP membranes (for 0.5, 1 and 2 wt. % hollow silica) and was compared with that of the pristine HEC membrane. The permeability values were calculated using the data obtained and computed with equation (1) for the tested gases and are tabulated in Table I. The selectivity of the various gases was studied by the given equation and the results shown in the Figure 4. As per the plot the highest permeability is shown for 2 wt. % silica loaded hydroxylethyl cellulose membrane i.e. 11,250 barrer for CO2. This shows that the modified membranes were proven to prefer permeation for CO2 gas. The same trend was followed for all the modified nanocomposite membranes i.e. for 0.5, 1 and 2 wt. % silica loaded HEC membranes. The lowest permeability was obtained for nitrogen gas starting from 950 to 1780 barrer.
Table1: Permeability data for the nanocomposite membranes
Hollow Silica NP’s
|
Permeability
|
N2
|
O2
|
H2
|
CH4
|
CO2
|
0
|
950
|
1780
|
3970
|
1790
|
6700
|
0.5
|
1010
|
2150
|
4120
|
2010
|
7130
|
1
|
1240
|
2490
|
4870
|
2510
|
8720
|
2
|
1780
|
3380
|
6430
|
3620
|
11250
|
Permeability is in unit of Barrer (1 Barrer = 10−10 cm3(STP)cmcm−2 s−1 cmHg−1).
Selectivity
The selectivity was calculated using Equation (2). The result of addition of small amount of the filler and slight increase in the filler content on the base membrane matrix for the permeability of CO2, CO2/N2 and CO2/CH4 selectivity of hydroxylethyl cellulose membranes consists of 0 to 2 wt% of SiO2 is shown in Figure 4. Compared to the pure hydroxylethyl cellulose membrane, the CO2, N2 and CH4 permeabilities of the modified membranes are now initially being reduced. The result of increasing the nanoparticle as filler content on H2 permeability, CH4/N2 selectivities of hydroxyl ethyl cellulose nanocomposite membrane containing 2% wt hollow silica nanoparticles is shown increasing curve slightly.
Figure 4: Selectivity data for various combinations of gases.
Figure 4 gives the clear picture about the membrane performance in terms of selectivity. The highest selectivity value is shown for CO2/N2 gas mixtures i.e. 6.3 to 7.1. The rest of the gaseous mixture viz., O2/N2, H2/N2, CO2/N2, CO2/CH4 and H2/CH4the selectivity decreased due to reduction in partial pressure gradient and due to coupling effect. This effect is explained clearly in two different ways, one is the plasticization effect of the membrane. Secondly the hollow silica adsorbs the gaseous molecules and fails to desorb the same.
Conclusions
The current research work which demonstrates the enhancement of gas separation properties of the synthesized nanocomposite membranes compared to the pristine hydroxylethyl cellulose membrane. The hollow silica embedded hydroxylethyl cellulose membranes have been measured for the different gases. The membranes were CO2 selective as compared to N2. Permeability of CO2 increased, but selectivity decreased with increasing the pressure in feed. Nevertheless, a small disparity in the kinetic diameter of the CO2 molecule yield in noteworthy differences in one of the variable, membrane selectivity; almost certainly it is judged. In extension, the results of the present study are a clear witness in the enhancement of the membrane performance because of the addition of small amount of hollow silica nanoparticles into hydroxyl ethyl cellulose for both the mixtures chosen in this study. In case of selectivity modified membranes fails to enhance the selectivity and this is attributed due to the plasticization effect of the membrane.
Acknowledgements
Authors are grateful to the B.V.V Sangha, Bagalkot for providing the research facilities and the Principal, Basaveshwar Science College, Bagalkot for his continuous support.
Funding Source
The author(s) declare(s) that the funding is done by author only.
Conflict of Interest
The authors declare that there is no conflict of interests regarding the publication of this article.
References
- R.Surya Murali, T. Sankarshana, S. Sridhar. Air Separation by Polymer-based Membrane Technology. Sep. Purif. Reviews. 2013;42:130-186.
CrossRef
- R. Surya Murali, S. Sridhar,T. Sankarshana, Y. V. L Ravikumar. Gas Permeation Behavior of Pebax-1657 Nanocomposite Me.mbrane Incorporated with Multi-walled Carbon Nanotubes, Ind. Eng. Chem. Res. 2010;49: 6530-6538.
CrossRef
- S. Sridhar, B. Smitha, T. M. Aminabhavi, Separation of carbon dioxide from natural gas mixtures through polymer membranes-A Review.Sep and Purif Rev. 2007;36:113-174.
CrossRef
- L. Deng, T. J. Kim, M. Sandru, M.B. Hägg. PVA/PVAm blend FSC membrane for natural gas sweetening. In: Hassan EA (ed) Proceedings of the 1st annual gas processing symposium. Elsevier, Amsterdam. 2009;1:247–255.
CrossRef
- M. D. Donohue, B. S. Minhas, S. Y. Lee Permeation behavior of carbon dioxide-methane mixtures in cellulose acetate membranes. J. Membr. Sci. 1989;42(3):197–214.
CrossRef
- J. D. Wind, D. R Paul, W. J Koros. Natural gas permeation in polyimide membranes. J Membr Sci. 2004;228(2):227–236.
CrossRef
- J. D. Wind The effects of crosslinking chemistry on CO2 plasticization of polyimide gas separation membranes. Ind Eng Chem Res. 2002;41(24):6139–6148.
CrossRef
- R. T. Adams R. T. CO2–CH4 permeation in high zeolite 4A loading mixed matrix membranes. J. Membr. Sci. 2011;367:197–203.
CrossRef
- R. W. Baker. Natural gas processing with membranes: an overview. Industrial & Engineering Chemistry Research. 2008;47(7):2109-2121.
CrossRef
- F. G. Kerry. Industrial Gas Handbook: Gas Separation and Purification. CRC Press,(2007).
CrossRef
- P. Bernardo, Membrane gas separation: A review/state of the art. Industrial & Engineering Chemistry Research. 2009;48(10):4638-4663.
CrossRef
- W. Schell. Recent advances in cellulosic membranes for gas separation and pervaporation. Gas Separation & Purification. 1989;3(4):162-169.
CrossRef
- S. Sridhar, R. S. Veerapur, M. B. Patil, K. B. Gudasi, T. M. Aminabhavi. Matrimid polyimide membranes for separation of carbon dioxide from methane,J. Appl. Polymer Sci. 2007;106:1585-1594.
CrossRef
- W. J. Koros, G. K. Fleming. Membrane-based gas separation. J. Membr. Sci. 1993;83:1– 80.
CrossRef
- P. R. S. Braga. Liquid phase calorimetric-adsorption analysis of Si-MCM-41.
- Evidence of strong hydrogen-bonding sites, Microporous Mesoporous Mater. 2011;139:74-80.
CrossRef
- Vadia. Mesoporous material, MCM 41: A new drug delivery. Asian J. Pharm. Clin. Res. 2011;4:44-53.
- D. M. Marzouqa, M. B. Zughul, M. O. Taha, H. A. Hodali, Effect of particle.
- morphology and pore size on the release kinetics of ephedrine from mesoporous MCM-41, materials. J. Porous. Mat. 2011;19:825-833.
CrossRef

This work is licensed under a Creative Commons Attribution 4.0 International License.