Various Material Development Strategies for Suitable Catalysts of Photo Catalytic Water Splitting to Green Fuel H2:A Critical Review
Introduction
The high energy crisis, fossil fuels depletion & environment pollution becoming the major global challenges in recent and upcoming years 1. Due to global warming& pollution issues, today’s world is searching for a sustainable, clean, and environment friendly alternatives. With a vast study on fuel and its negative impacts, scientists came to know that, hydrogen fuel may be a solution for this crisis. H2 is a fantastic energy conveyer with a high CV and clean property. As a bountiful element, H2 appear in many substances in the earth likely seawater, Biogas, freshwater and fossil fuels, etc. For the production of H2 with zero or low impact on the environment, almost all Carbon-dioxide with other unwanted particulates should be separated when H2 is produced from fossils 2. Till now, hydrogen is mainly synthesized via steam reforming method where CO2 is formed simultaneously. And this CO2 is the key concern for today’s environmentalists, as it is the major greenhouse agent. Photo-catalytic water splitting may be a solution to these problems. Some other important sources of the hydrogen production process are Electrolysis, Thermochemical processes, Dark fermentation process, Thermolysis, Photo-fermentation or Bio-photolysis, Hybrid thermochemical cycles, and Photo-catalytic water splitting, etc. For ultra-pure H2 production, electrolysis is a more reliable, technically sound, and widely acceptable system. Electrolysis is a technique where direct current (DC) is used to drive the anion-spontaneous electrochemical reaction 3. To run this technique, immaculate power hubs may be engaged like as solar-powered energy, geothermal based energy, biogas energy, wind-powered energy, ocean and others. The energy produced from nuclear energy sources may also be used for driving this process. Hydrogen produces in this technique is purer than any other technique, O2 production was also high in this technique. But the major disadvantage of this technique is that a huge amount of electricity is needed to run the electrolysis technique and the initial set-up cost is expensive in amount. The major reaction involve with the electrolytic technique of water is generally written as:

On the other hand, thermochemical processes are another convincing process for hydrogen production. The thermochemical system is the process which mainly driven by heat as its primary energy. This type of process for the production of hydrogen is considered as the viable process for the probable alternative of the previous one. Most alluring support from thermochemical production cycles is no requirement of catalysts or agents to drive the process reactions 4. All chemicals, without water (main raw material), used here can be reused easily. Other mesmerizing support of these types of degradation process are: (a) H2 and O2 can be separate from mixture gas without membrane; (b) optimum energy requirement range of 327–927 oC; & (c) less electrical power consumption. This requirement of power can be provided by concentric solar energy, the geothermal energy, biogas, nuclear electricity, and other sources5. But the challenges of this technique is that the bulk amount of heat supply source is not available in everywhere and artificially generation of heat is also costly. Here alleviate temperature degradation of catalyst is a major challenge, which retard the hydrogen production rate.
On the other hand, thermolysis is another significant way of electrolysis where H2O is split to H2 and O2 at elevated temperatures ranging between 973K to1273K. Basically this process is more effective than generally used room-temperature splitting process where efficiency increases with the increment of heat. The electrical energy needed here is much less than the conventional methods. Zero GHG emission is possible here by using the clean source of energy like solar, geological, wind or nuclear (6).Heat recovered from another process (such as industrial boiler or heat exchanger) can also be effectively used to run this process (7).In high temperatures operating system, the process units must have met some definite requirements for effective H2 production. Most recent objections of these types of the process can be enlisted as: (a) stable electrolytic reagent synthesis with low electronic and strong ionic conductivity; (b) porous, low cost and chemically static electrode development for high electronic conductivity and finally (c) mechanically stable engineering materials at high temperatures. Again, Hybrid thermo-chemical cycles which conduct at low temperature is more viable while comparing with the thermally driven water-splitting system. Electrical and thermal energies fulfill the outer energy demand of particular reactions as hybrid systems conducted at lower temperatures. A major advantage of these types of systems is that H2 generation from low-grade energy sources. But the major findings of this technique are that bulk amount heat source is not available everywhere and artificial generation of heat is also costly.
Bio-photolysis as well as photo-fermentation techniques are familiar as photon-based biochemical H2 generation from water. The main privilege of this technique is the capability to generate H2 from H2O in aqueous media at conditioned temperature & pressure 8. Here in the Bio photolysis processes light-sensitive micro-organisms are used as biological converters in a special bioreactor. Here Microalgae are most preferable among all microbes because they are culturable in suitable media 9. Cultured microalgae isolates have the capacity to produce H2 in a closed system and they show high H2 yields. The primary hydrogen generation reactions using photo-activated enzymes are the following:
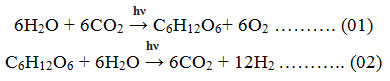
However, this process is demonstrated at laboratory scale only and piloting is not yet possible to support the industries at the commercial level. Again Dark fermentation is a fermentation process which defined as the transformation of biochemical energy of organic to different forms of energy in dark conditions (during the reduced supply of light). In this process biochemical energy available in organic matter used to obtain H2 in absence of light 10. Bioreactors used in these types of fermentation are cheaper and simpler in comparison with photo-fermentation because this method does not need solar input processing. There are several other benefits of H2 production by dark fermentation like, 1 H2 production from organic waste and (2) stabilized & control in bio-waste for reducing potential danger of pollution 11. The dark fermentation process can be implemented in water treatment units for H2 production from organic wastewater. H2 production costs can be reduced by using inexpensive and readily available organic wastes (including wastewater). But the major disadvantage of this method is that the hydrogen production rate is very low and huge amount of reagents are required for continuous fermentation process which are non-recyclable.
From all available H2 production the process discussed here photo-catalytic water splitting using sunlight has been widely believed as a promising process for green and environment-friendly hydrogen generation, where a chemical reaction occur while following with the alteration of Gibbs free energy (Eq. – 3)12

This type of Water Splitting has harmony with photosynthesis because both of them are uphill type. From this site, the water-splitting system can be evaluated as artificial photosynthesis. The utilization of green, inexhaustible and sustainable solar energy is an urgent need to avoid the greenhouse effects. A huge amount of research articles are circulating every year on solar energy transformation systems like photo-catalytic conversion of organic pollutants, hydrogen (H2) gas production through photo-catalytic water splitting, photovoltaic cells, and dye-sensitized solar cells 13. Photo-electrochemical water splitting process by Fujishima & Honda in the year of 1972 is most variant of this technique 14. Following this way, till now H2 production by Photocatalytic water splitting is considered as a promising technology for green energy revolution 15-17. (TiO2)titanium oxide 18-20, (g-C3N4)Graphitic-Carbon Nitride 21-23 and (CdS) Cadmium Sulfide (24-26)are three widely studied catalysts for water splitting in the last few decades. Among them, titanium oxide (TiO2) is found to be more superior, and a benchmark photo-catalyst still now 27.
Figure 1: Photo-catalytic H2 productionusing suitable semiconductor 28.
Due to its photostability, efficiency, band edge, non-toxicity and sustainity it became a burning topic in this sector 29-34.The mechanism of Photocatalytic activity of TiO2 based catalyst for water splitting are illustrated in Fig. 1.0 35. The overall water splitting reaction on a photo-catalyst comprised of the following half reactions:
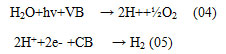
Overall Reaction:
:
Herein, an overview of current development in photocatalyst, a comparison of different water splitting processes, various catalyst development techniques especially doping on composite matrix, its effect on hydrogen production and various types of doping technique are critically explained. Different techniques for catalyst development including doping, Heterojunction, Dye sensitization, etc. are explained here. A complete study on doping is discussed here from all of its aspects. The numerous factors that affect the photo-catalytic water splitting such as band gaps, morphology, temperature, light intensity, pH, oxygen vacancies, and activity of sacrificial reagents are then scrutinously discussed here. Additionally, the reactor phenomenon and their commendation to further modification for H2 production are also explained here.
Advancement in photo-catalyst developments
As per characteristics of elements throughout periodic table, a good number of semiconductors have shown the capability for photo-catalytic water splitting to produce hydrogen. The requirements for a photocatalyst to be viable on large scale usage involves high quantum yield; being inexpensive, earthly abundance, recyclable, nontoxicity, resistant to corrosion, and photo-stable; and also must have a long lifetime 36. TiO2, since Fujishima and Hondas study at 1972, has been regarded amongst the most preferable photo-catalysts for splitting water for its conveniences, e.g. being abundant, inexpensive, noncorrosive, photo-stable, and environmentally friendly(14). Nevertheless, TiO2 can use only UV light for H2 evolution for its vast band-gap energy of 3.2 eV, separated electrons and holes in VB and CB of TiO2 can swiftly recombine and release energy or useless photons that barricade its practical utilization for Photocatalytic H2 generation using solar energy 37.Solar energy utilization basically depends on the electromagnetic wavelength of radiation in which photocatalyst shows activeness. Within 400 nm, the conversion efficiency of solar energy does not reach beyond 2% 38. Efficiency improves to 16% and 32% if the spectral wavelength reaches 600 and 800 nm respectively. Oxides other than TiO2, e.g. Cu2O, WO3, Fe2O3, and BiVO4, were also examined for photo-catalytic H2 production, but their photo-catalytic efficiency for hydrogen evolution is still restricted by small amount of light-harvesting, large electron-hole recombination rates, and poor transportation of charge 39. In times of BiVO4, its edge position is inconvenient for HER. For Cu2O, the efficiency solar to energy conversion is 18.0% theoretically, and it is vulnerable to auto-reduction in aqueous medium 40.That’s why it is very important to develop new photo-catalysts to use the visible range and near IR region wavelength. Metal-free and Earth-rich catalysts such as g-C3N4, graphene, oxide of graphene, carbon nano-tubes have been examined greatly owing to its possible cost minimization in the time of scaling up 41. In recent times g-C3N4 based materials free from metal have gained popularity for application in splitting of water 42. However, the catalyst g-C3N4 still suffering from many drawbacks, for instance insufficient surface morphology, reduced conductivity & large recombination rate 43. Another significant catalyst named metal-organic frameworks or MOFs are excellent photo catalyst for photo catalytic H2 generation 44. MOFs are composed of inorganic ions and organic substrate, which function as linking point & as connectors, correspondingly 45. At last Researcher heed their concentration on catalyst development by implementing different technique such as Doping, Nanostructure, Heterojunction, Dye sensitization, Formation of Solid Solutions, Sensitization with noble metal and Increasing Photo catalytically Active area etc.46. Amid all of the technique applied Doping and Heterojunction is more striking.. Table 01 gives the recent update on catalysts with various morphology & photocatalytic H2 production efficiency47.
Table 01: Recent updates in photo-catalysts with different morphology and photocatalytic efficiency.
Catalyst |
Morphology
|
Synthesis method
|
Source of irradiation
|
H2 generation rate
|
Ref.
|
Ag/TiO2, Hg |
nanoparticle
|
sol-gel
|
Vapor lamp (365 Nm)
|
910 mmol g1 h1
|
(48)
|
Ni/TiO2, Pyrex |
nanosheets
|
solid state reaction |
F lamp, 100 W (365 Nm) |
26,000 mmol g1 h1
|
(49)
|
Fe3+ ions doped and Ag deposited TiO2 |
nanoparticle
|
solvothermal |
UV light Source |
515.45 µmol/h/g
|
(50)
|
Fe/ TiO2 |
Na
|
anodization |
xe arc lamp
|
174.30 mmol g1 h1
|
(51)
|
Cu/ TiO2 |
nanotubes
|
anodization |
300 W, xenon,lamp |
28,700 mmol g1 h1
|
(50)
|
Au/ TiO2 |
Na
|
anodization |
300 W, xe lamp
|
3550 mmol g1 h1
|
(52)
|
Cu/ TiO2 |
nanoparticle
|
commercial tio2
|
125 W, Mercury Vapour lamp ( High Pressure)
|
01–07 µmol/min
|
(53)
|
Pt or Au/TiO2 |
nanotubes
|
anodization |
Xe/Hg lamp ( High Pressure)
|
.06 µmol/cm2/h
|
(54)
|
Au/Ti0.9O2
|
nanosheets
|
solid state reaction |
xe lamp 300 W
|
6753.00 µmol/h/g
|
(55)
|
TiO2/WO3/Au |
Na |
electrospinning
|
Xe Arc lamp,300 W
|
270 µmol/h
|
(52)
|
rutile TiO2
|
nanosheet
|
solvothermal
|
300 W, xenon lamp
|
22 mmol/g/h (5 h)
|
(56)
|
N/TiO2, PdO & Pt loaded NTiO2
|
nanoparticle
|
sol-gel
|
400 W, mercury lamp
|
55.0,544.0,772.0 µmol/h/g |
(48)
|
SMK-TiO2 |
nanoparticle
|
sol-gel
|
150 W Mercury lamp ( Medium Pressure)
|
3.3 mmol/240 min
|
(57)
|
Co-moderated TiO2
|
nanoparticle
|
commercial TiO2
|
300 W, Xenon lamp
|
Nanoparticle
|
(58)
|
Ag-Fe/TiO2 |
nanotubes Ti sheet(> 99% purity) |
electrochemical
|
Xenon Lamp& Hg Llamp
|
Nanotubes Ti Sheet(> 99% Purity) |
(53)
|
Cu(OH)2/TiO2
|
nanotube arrays
|
electrochemical anodization
|
300W, Xe lamp
|
Nanotube Arrays
|
(59)
|
Material (Catalyst) Development techniques
Most decisive characteristic of a photocatalyst are: its optimum band gap, surface morphology, crystal structure and stability under light irradiation..TiO2 based photocatalyst orAnatase TiO2 is the photocatalyst mostly subjected to modification techniques to enhance H2 production. Other oxides, e.g. g-C3N4, Cu2O, WO3, Fe2O3, and BiVO4, were also studied for photo-catalytic H2 production, rather their performance or efficiency for photocatalytic H2 evolution is evaluated by some factors like, light harvesting time and rate, charge recombination, band edge and low charge transportation 60. Also, catalysts such as g-C3N4, oxides of graphene, graphene, nanotubes, and CDQs have been extensively investigated to develop their properties as they can exhibit better efficiency 61 . Scientists are trying to develop the catalytic activity of photo-catalysts through several techniques. Some of these are
Catalyst Development techniques
Doping
Heterojunction
Dye sensitization
Sensitization with noble metal loading
Increasing Photo catalytically Active Area
Modification of surface
Nanostructure
modification with Co-catalysts,
Doping
Among all of the stable and effective techniques for the development of catalysts, doping is the most effective and widely applied way to gear up catalyst’s electronic activity as well as to accelerate the reaction surface. Non-metals and metals deposition onto the semiconductor surface enables supplying maximum active sites excellently for photo-catalytic hydrogen production. Besides, proper control of homogeneous distribution and metals particle size can supply sufficient active site resulting in maximum light transmittance 45. Catalyst modification induces more charges due to the reduction of band gap, hence exciting more electrons for the production of hydrogen 61. TiO2 is one of the prominent and pioneer catalyst with maximum efficiency from 1972 to now. Here, metal and non-metal doping of TiO2, elemental doping on titanium-based catalyst, doping on the various composite catalyst, such as g-C3N4, BiVO4, MoS2/Bi2S3, BiFeO3 are discussed in a brief.
Elemental doping
Doping deals with the replacement of an anion or cation within the surface of a substrate with some other agents(element) (Fig. 2a). Only an element of a similar charge and radius can be placed into a cation. The doping technique affects the band structure and decreases the band-gap by introducing a new forbidden level in the band. To choose the metal, its radius must match with the radius of the ion to be replaced. For example, in SrTiO3 base lattice, Ti4+ (0.0605 nm) will be replaced by Ta5+ (0.064 nm) and Cr3+ (0.0615 nm) ions where F (0.133 nm) atom will replace by O2 (0.140 nm) 62.
Figure 2: A 3-Dimensional representation for elemental doping on SrTiO362
Doping with single element
Single-element doping is the replacement of single sites with one similar type of foreign element (fig. 2a). Although the sites are normally cation sites in the perovskite structure, such as A & B, but most replacement occurs at B site (fig. 2b). Different effects on PEC materials will occur for each doping entity because of the difference in the arrangements of valence shell electrons. Primarily, new donors or acceptors cause to form by doping in the forbidden band, resulting in the reduction in band gap. Currently, Cu doped CaTiO3 shows an excellent result in H2 production with a rate of 1447.8 mmol h-1 under irradiation of UV light 63. Here, table 02 exhibits the expected improved data obtained from photo catalyst while doping with a different single atoms with variable parameters. Results incur that the CaTiO3 doping with Cu shows highest H2 evolution rate of 1447.8 mmol h1 with UV light 64. According to Shen P Et. al. SrTiO3 catalyst, synthesized by polymerization method yield 47.00 mmol h1 H2 evolution rate under λ>420.0 nm & λ63. On the other hand according to the study of Sun X Et. al. Sr2TiO4 exhibit maximum hydrogen evolution i.e. 97.7 mmol h1 when doping with Cr under light irradiation of (λ>250 nm, visible) 65. They developed this catalyst with Solid-state reactions mechanism and found highest output. Zou J-P Et. al., Yu H Et. al. get a regular output from SrTiO3 catalyst while doping with Zn and Cr respectively under UV light irradiation 66, 67. They synthesized the catalyst matrix in Sol-gel method. However, a significant result was found by Zhang HEt. al. from the catalyst Ti0.98Cu0.02O3 by Ca doping system under UV light irradiation. Zhang HEt. al. found that 1447.8 mmol h1 hydrogen evolution from catalyst while doping with Ca with Sol-gel method 64.
Table 02: Outcome of doping with single element at perovskites (titanate-based) structure.
Results obtained by single-element doping to titanate-based perovskites
|
No |
Catalyst |
Dopants |
Method |
Light source |
H2 generation rate |
Ref. |
1 |
SrTiO3 |
Rh |
polymerizable complex, |
UV lamp |
47.00 mmol h1 |
(63)
|
2 |
Ti0.98Cu0.02O3 |
Ca |
sol-gel |
UV lamp |
1447.80 mmol h1 |
(65)
|
3 |
Sr2TiO4 |
Cr |
solid-state reactions |
visible |
97.70 mmol h1 |
(66) |
4 |
Ba5/6/6TiO3 |
Zn |
sol-gel |
UV lamp |
2.90 mmol h1 |
(66)
|
5 |
Sr2/3TiO3 |
Zn1/3 |
sol-gel |
UV lamp |
12.10 mmol h1 |
(64)
|
6 |
SrTiO3 |
Cr |
hydrothermal |
visible and UV lamp |
9.30 mmol g1 h1 |
(67)
|
Doping with double element
Here, replacement in the host material cause to happen with two types of foreign substrate at the same sites (B) or separate sites like X sites and A, B. Below in table 03, few effective cases listed through doping with the double element, along with the used parameters in experiments are shown. For example, on doping of cation with two elements, Sun et al. reported the increased hydrogen evolution rate to 211.4 mmol g1 h1 only with visible light (l > 415 nm) in SrTiO3, Ta5+ and Cr3+ at Ti4+ sites compared to 97.7 mmol h1 H2 evolution rate for doping of SrTiO3 with Cr. High improvement, above two-fold, in case of Ta addition 65. Furthermore, the reported rate of H2 evolution can be more accelerated if UV light source is included. On Co-doping, diffuse reflectance spectra revealed that Cr/Ta ions form a 3d donor level of Cr3+, above O 2p valence band. Yu et al. reported similar results, as in table 3, Yu Het. al. shows. A study shows that the rate of hydrogen evolution in a double element doped SrTiO3 was more about twice than that of mono element doped SrTiO3, while fabricated through the same method. For B 2p electron, top valence band position was around 0.38 eV 67. But as Kang & Parkreported the performance of composite catalyst material doped with double-element (15.4 mmol h1) was less than that of (Cr, Ta):SrTiO3. 68. Results reveals that application of double doping in cation sites shows best outcomes than doping with only single element.
Table 03: Outcome of doping with double element at perovskites (titanate-based) structure
Outcomes of titanate-based perovskites listed through doping with double element
|
No.
|
Catalyst |
Dopant |
Method |
Light source |
Rate of H2 generation |
Ref. |
1
|
SrTiO3 |
Ta, Cr |
spray pyrolysis |
λ>415 nm |
211.4 mmol g1 h1 |
(68) |
2
|
Sr0.9Bi0.1Ti0.9 |
Fe0.1O3 |
solid state |
λ > 250 nm |
185mmol g1 h1 |
(69) |
3
|
SrTiO3 |
B, Cr |
hydrothermal |
visible & UV |
15.41 mmol g1 h1 |
(67) |
4
|
CaTiO3 |
La, Ag |
sol-gel with ultra-sonication |
visible & UV |
1064mmol g1 h1 |
(70) |
5
|
SrTiO3 |
Al, Au |
solid state |
visible & UV |
348 mmol g1 h1 |
(71) |
Doping with triple element
In doping with triple element, three dopant elements replace original host structure atoms, where two replace at A and B sites and the left other replaces O sites. Researchers doped anion to substitute O2 with F for performing a research on material doped with double element in the same material as that used byKang HW et. al. in his work. Resultantly, for maintaining balance in charge, rest elements changed their charge in the initial structure. For instance, in case of SrTiO3:Cr/Ta , when F ions substitute O2 ions Rh4+ & Ti4+ reduces Rh4+ respectively to Rh3+& Ti3+and it partially induces Rh3+ and Ti3+ formation, respectively from Rh4+& Ti4+ in the sites B of the substrate, decreasing barrier towards the energy of activation and reducing stability 68. Here, charge distribution variation influences the band organization through making interactivity within electrons in a substrate. Also, F replacement in O2 sites results in cation deficiency and cation deficiency are the root cause for the doping element to improve H2 evolution in PEC. In a research by Kang HW et. al. they noticed that catalyst SrTiO3 yield 123.7 mmol g1 h1 H2 while doping with tri–element such as (Rh/Ta/F). They synthesized the catalyst with Spray pyrolysis method and the light irradiation was 4% under visible range. At the same time in a separate investigation, by Kang HW et. al. registered that 3887.9 mmol g1 h1 hydrogen was evaluated from theSrTiO3 catalyst while doping with Cr/Ta/F simultaneously.They also fabricate the catalyst matrix by spray pyrolysis method and conducted the reaction under visible light irradiation 71.
Table 04: Outcome of doping with tri-element at perovskites (titanate-based) structure
Outcome of perovskites (titanate-based) listed through doping with tri-element
|
No |
Catalyst |
Dopants |
Fabrication Method |
Wave Nature |
Rate of H2 Production |
Ref. |
1 |
SrTiO3 |
F/Ta/Rh |
spray pyrolysis |
visible range |
123.70 mmol g1 h1 |
(71) |
2 |
SrTiO3 |
Ta/F Cr |
spray pyrolysis |
visible range |
3887.5 mmol g1 h1 |
(62) |
Doping on photo-catalysts based on Graphitic carbon nitride (g-C3N4)
Doping with metal
Recently Graphitic carbon nitride(g-C3N4), drawn a considerable concern as well as effectively investigated just as green, viable photo catalyst which results in a convenience e.g. metal-free, non-toxic semiconductor along with sensibility at the visible light range and narrow band gap , approximately, 2.7 eV 72. In addition, consistingwith simple earth abundant element C & N, it is of low cost and also shows unique resistivity towards photo corrosion for the strength of covalent bonds shown by the atoms of carbon and nitride 73. Also g-C3N4 shows exceptional photoluminescence (PL) characteristics, making it unstable as quality co-catalyst for other semiconductor catalysts 74. But in practice, implementation of g-C3N4 as photo catalyst is limited, due to smaller specific surface area and high rate of recombination of photo induced charges 75. Ultimately, Doping is one of the effectual initiative for adjusting electronic formations of g-C3N4 and to stimulate the reaction surface to escalate photo-catalytic activity owing to the work function and Surface Plasmon Resonance effect according to the previous writings 76.
Figure 3: Metal doping on g-C3N4 based photo
In the year 2014,Zhong Y et. al. conduct Pt doping on photo-catalysts based on Graphitic carbon nitride (g-C3N4), under light irradiation of (300 W Xe lamp, l >420 nm) in Quartz reactor. They get hydrogen flow rate of 41.7 μmolh−1g−1 which was not significant to continue further work. Further in 2015 prominent researcher Huang Z et. al and Ma L et. al. again dopedg-C3N4 photo catalyst with Pt . The final product was then led for photoreaction under light irradiation of 300 W Xe lamp, l >420 nm in Quartz reactor and Pyrex reactor respectively 77, 78. Ma L et. al. found89.28 μmolh−1g−1 hydrogen production rate while Huang Z et. al. found 261.8 μmolh−1g−1in Quartz reactor. In 2018, Wang Y et. al doped potassium (K) on Graphitic carbon nitride (g-C3N4) based photo-catalysts. They found that, in Pyrex reactor under light irradiation condition of 300 W Xe lamp, l ¼ 400 nm, K doped g-C3N4 produce maximum 1337.2 μmol hydrogen at one hour 79. In the same year, another study of Liu M et. al. shows maximum 4210.8 μmolh−1g−1 efficiency of Graphitic carbon nitride (g-C3N4) based ,while doping with platinum (Pt) 80. But in 2019, Chen D et. al. found 79.00 μmol hydrogen by Mo doping on Graphitic carbon nitride (g-C3N4) based photo-catalyst 81.
Table 05: Metal doped photo-catalysts based on g-C3N4 for H2 production.
Year |
Photocatalyst |
Dopant
(metal) |
Reactor |
Light Source |
Efficiency
μmolh−1g−1 |
Ref. |
2019 |
g-C3N4 |
Mo |
Pyrex reactor(P) |
Xe lamp, 300 W |
790.0 |
(81)
|
2018 |
g-C3N4 |
Au |
Pyrex reactor(P) |
Xe lamp, 300 W |
265.00 |
(82)
|
2018 |
g-C3N4 |
Cu |
Pyrex reactor(P) |
Xe lamp, 300 W, l >430 nm |
3020.00 |
(83)
|
2018 |
g-C3N4 |
Ni |
Quartz reactor (Q) |
,Xe lamp, 300 W, l >430 nm |
529.10 |
(84)
|
2018 |
g-C3N4 |
Ni-P |
Pyrex reactor(P) |
Xe lamp, 350 W |
937.00 |
(85)
|
2018 |
g-C3N4 |
Pt |
Pyrex reactor (P) |
Xe lamp,350 W |
4210.80 |
(80)
|
2018 |
g-C3N4 |
K |
Pyrex reactor (P) |
Xe lamp, 300 W l ¼ 420 nm |
1337.20 |
(79)
|
2017 |
g-C3N4 |
Co |
Pyrex reactor(P) |
Xe lamp, 300 W, l > 420 nm |
280.00 |
(86)
|
2017
|
g-C3N4 |
Pt |
Pyrex reactor(P) |
Xe lamp, 300 W, l ¼ 400 nm |
740.00 |
(87)
|
2015 |
g-C3N4 |
Pt |
Quartz reactor(Q) |
Xe lamp, 300 W, l >420 nm |
262.00 |
(88)
|
2015 |
g-C3N4 |
Pt |
Pyrex reactor (P) |
Xe lamp, 300 W l >400 nm |
890.20 |
(78)
|
2014 |
g-C3N4 |
Pt |
Quartz reactor (Q) |
300 W,Xe lamp, l >420 nm |
420.00 |
(77)
|
Non-metal Doping
Besides metal doping, doping with non-metals can effectively increase semiconductor’s photo-activity. In the modification of g-C3N4 polymers, doping with O, S, B & N; (the non-metal elements) shows excellent result while comparing with others. Its optical & electronic nature were effectively optimized for promoting photo-catalytic activity as a result of improved optical absorption process along with increased charge mobility. Hydrogen gas evolution performance should be enhanced as loading of various type of non-metal element that tunes the catalyst formation by reducing the band gap, inhibiting electron-hole pair recombination rate and stabilizing the catalyst. Zhou et al., during 2016, reported near 3.3 time’s higher hydrogen production than undoped g-C3N4 over modified N-doped g-C3N4 photo-catalyst (CN-20) with maximum reported hydrogen production was 64 mmol h-1 (Fig. 6(a)) 89. CN-20 catalyst was also documented for stability, as there was no notable decrease in the hydrogen production rate on repeated experiment under similar experimental situation used for four cycles (24 h), as Fig.6(b) reveals.
Figure 4: Non-metal doping on g-C3N4 based photocatalyst
In another study by Chen et al. reported the 12 times higher enhanced photo-catalytic performance for sulfur loading than pristine g-C3N4 and long term stability for the water-splitting process, as it is documented in Fig. 6(c). This one resolved that S loading is able to efficaciously enhance the specific surface area, persuade the formation of nitrogen vacancy, retard photo produced electron-hole pair recombination rate and improve the visible range responsiveness of the catalyst 90. Similarly specified the non-metal Br doped g-C3N4, was also appeared having long-term stableness on visible range photo-catalytic activity for 20 h with highest hydrogen generation rate of around 48 mmol h-1 as in the Fig. 6(d) showed. They also suggested that, the mechanism of reaction for water splitting using photo catalyst designed for CNBr as demonstrated in the Fig. 6(e). 91. Here, Table 7 demonstrates the cases for the non-metal doping on g-C3N4 aimed at water splitting using photo catalyst.
Table 06: The recent study of Non-metal loading on photocatalyst based on g-C3N4 for hydrogen generation.
Year |
Photocatalyst |
Dopant
(inorganic) |
Reactor |
Light Source |
Efficiency
μmolh−1g−1 |
Ref. |
2019 |
g-C3N4 |
S
|
Pyrex reactor (P) |
Xe lamp,300 W,λ = 420 |
1511.2 |
(92) |
2019 |
g-C3N4 |
P
|
Pyrex reactor(P) |
350 W, Xe arc lamp, λ > 420 nm |
2020 |
(93) |
2018 |
g-C3N4 |
O
|
Pyrex reactor(P) |
300 W, Xelamp λ = 420 |
1968.00 |
(94) |
2018 |
g-C3N4 |
B/F
|
Pyrex
reactor(P) |
Xe lamp, 300 W, , λ> 420 nm |
6870.00 |
(95) |
2018 |
g-C3N4 |
S
|
Pyrex
reactor(P) |
Xe lamp, 300 W, λ> 420 nm |
240.1 |
(84) |
2018 |
g-C3N4 |
O
|
Pyrex reactor(P) |
Xe lamp, 350 W, |
1968.00 |
(94) |
2017 |
g-C3N4 |
P
|
Pyrex reactor(P) |
Xe lamp, 300 W, λ > 420 nm |
570.00 |
(94) |
2017 |
g-C3N4 |
C
|
Pyrex reactor(P) |
Xe lamp, 300 W, λ> 420 nm |
807.40 |
(96) |
2016,
|
g-C3N4 |
Br
|
Pyrex
reactor(P) |
Xe lamp, 300 W, λ ¼ 400 nm |
480.0 |
(91) |
2016 |
g-C3N4 |
N |
Quartz reactor(Q) |
Xe lamp, 300 W, λ > 420 nm |
640.0 |
(89) |
2015 |
g-C3N4 |
C |
Pyrex reactor(P) |
Xe arc lamp, 350 W, λ >420 nm |
540.0 |
(97) |
2014 |
g-C3N4 |
C |
Pyrex
reactor(P) |
Xe lamp ,Visible light, λ > 420 nm |
700.0 |
(98) |
Doping on BiVO4 based Photo-catalysts: BiVO4
Centenary of semiconductors are used for their Photo catalytic Properties, because it is impossible to fulfill all the conditions of photo catalytic water splitting by a particular element. Accordingly, element improvement stands the significant note for improving Photo catalytic water splitting act. In recent time, BiVO4 takes excessive consideration as photo-anode substances for PEC water splitting, since certain modified BiVO4 photo-anodes fulfill several essential necessities enlisted above 99-101. BiVO4 is a n-type semiconductor photo catalyst with the maximum band gap energy of 2.4eV. Here, BiVO4 engrosses sufficient amount of visible light spectrum and used as a stable, neutral, nontoxic, comparatively low-cost electrolyte 102-104. In comparison with other common O2 evolution photo catalyst likeFe2O3 and WO3, BiVO4 consumes a quite high CB (conduction band) energy (0.02 V vs. RHE). Therefore, the needless bias potential to elevate the photoelectrons upper the water reduction potential (0.0 V vs. RHE). However, the drawback is a very poor electrical conductivity with drippy water oxidation kinetics. Hence, several improvement techniques had undertaken for takeover the shortcomings of the BiVO4 catalyst as a photo-anode and to magnify its photo catalytic water splitting properties. Doping is one of the significant steps of them. Bismuth vanadate is suffering from the problems of the transfer of electron. Therefore, doping in BiVO4 can be a noble strategy to develop the electron transfer process in it and later photocurrent along with catalytic execution. In a research by Patil et al. informed that 2.00% of silver which is doping in uncontaminated BVO could rise its photocurrent density near about 3-fold as linked to an undoped BVO.In another work ,Fang and his team presented that, the pure BVO Catalyst have less photocurrent density rather than Ag-BiVO4 structure 105.
Where Martin Rohloff et al. described that the photo catalytic water splitting routine of the Mo-doped BiVO4 film photo-anodes are much better compared to their pure BiVO4. The photocurrent densities for Mo-BVO go to 1.90 mA cm−2 at 1.23V vs. RHE under solar light irradiation (100.00mW cm−2). In a work, Diane K. Zhong et al. described that the photo-current densities of BiVO4, after W doping were improved to 0.7 mA cm−2 at 1.23 V vs. RHE under 1.0 sun light illumination (100.00mW cm−2) 106. Amongst surface modification techniques deposition of catalysts layers on the BiVO4 surface, cobalt phosphate (Co-Pi) displayed the significant benefits of being effortlessly coconspirator with the BiVO4 surface. The Co-Pi alteration is a modest and effective technique to produce an earth abundant water-oxidation electro-catalyst for developing photo catalytic water oxidation 107. The modification of surface of BiVO4 semiconductor with W-doped and Mo-doped, are increased the photocurrent densities to 1.25 and 4.6 mA cm−2at 1.23 V vs. RHE under illumination of 1.0 sun light, respectively 103, 108. Heretable 07 shows the recent study of doping on BiVO4 composite photo catalyst.
Table 07: The recent study of doping on BiVO4 compositephoto-catalyst.
Year |
Photocatalyst |
Dopant
(metal) |
Reactant Solution |
Light Source |
Efficiency
μmolh−1cm−2 h−1 |
Ref. |
2018 |
BiVO4 |
Nb-TiO2
|
1.0M KCl at PH 9.2 |
AM1.5Gillumination |
80μmolh−1cm−2h−1
|
(109) |
2018 |
BiVO4 |
Bi2S3 |
Na2S/Na2SO3 |
1000 W m−2 (1 Sun). |
417 μmolh−1cm−2 h−1 |
(106) |
2018 |
BiVO4 |
Nb-TiO2/W |
0.50 M phosphate buffer |
AM1.50G illumination |
80 μmolh−1cm−2 h−1 |
(110) |
2017 |
BiVO4 |
MDH |
0.50 M phosphate buffer |
AM1.50 G illumination |
21 μmolh−1cm−2 h−1 |
(111) |
2017 |
BiVO4 |
3-Fe2O3 |
phosphate buffer solution |
AM1.50 G illumination |
27.34 μmolh−1cm−2 h−1 |
(112) |
2016
|
BiVO4 |
CDs |
0.50 M phosphate buffer |
300.0 W Xe-lamp |
0.92 μmolh−1cm−2 h−1 |
(107) |
2016
|
BiVO4 |
HDP |
1.0M KCl at pH |
1.0 G sun illumination |
121 μmolh−1cm−2 h−1 |
(107) |
2016
|
BiVO4 |
WO3-NRs |
potassium phosphate(pH =7) |
solar light, AM 1.50 G |
102 μmolh−1cm−2 h−1 |
(105) |
2014
|
BiVO4 |
WO3/(W; Mo) |
0.5M K2SO4
(pH 7.0) |
100mWcm−2 , AM 1.50 G, |
75 μmolh−1cm−2 h−1 |
(105) |
2011 |
BiVO4 |
RhO2/Mo |
– |
AM1.50 G solar light |
9 μmolh−1cm−2 h−1 |
(113) |
2011 |
BiVO4 |
Mo
|
seawater |
simulated AM 1.50 G sunlight |
7 μmolh−1cm−2 h−1 |
(113) |
Doping on BiFeO3 based photo-catalysts: by Gd3+
In a work,Yuxuan Yanget. al. reported that, sol-gel synthesis along with photo-catalytic H2 evolution of BiFeO3 and Gd3+ doped BiFeO3. The results of this work exhibits increased photo-catalytic H2 evolution due to Gd3+doping.The H2 production rates are about 21.90 and 67.60mmolcm-2h-1 for BiFeO3 and Gd3+ doped BiFeO3, respectively. So, it is absolutely larger photocatalytic activity, the rate, more than three times of Gd3+ doped BiFeO3 than that for pristine BiFeO3. So, Gd3+ doping effects performance of BiFeO3 114. Also, It is mentionable that for a particulate photo-catalyst the H2 production ability depends on its different properties e.g. microstructure, crystallinity, electronic and optical etc.115.
Table 08: Key findings in development of Photocatalytic activity of BiFeO3 by doping with Gd3+114.
Sl. No. |
Catalyst |
Doped
Element |
Preparation Method |
Efficiency |
Ref. |
01 |
BiFeO3 |
nil |
sol-gel synthesis |
21.9 mmolcm-2 h-1 |
Yuxuan Yang, et. al., 2018 |
02 |
BiFeO3 |
Gd3+ |
sol-gel synthesis |
67.6 mmolcm-2 h-1 |
Yuxuan Yang, et. al., 2018 |
Doping on TiO2& TiO2 composite photo-catalyst
TiO2 & TiO2 composite photocatalyst is the best, efficient, and cost-effective catalyst for photo-catalytic water splitting. Since 1972 enormous scientists or researchers work on it. The TiO2 & TiO2 composite photocatalyst could be further stabilized by the deposition of various metal and non- metal elements on its surface to increase catalytic activity. According to Hang Liu et. al. the photocatalyst Pt/PCN-777 exhibits excellent H2 production rate of 586 mmolcm-2 h-1in the presence of the TEOA reagent as a sacrificial agent under 300.0 W xenon lamp 116.The most important is that, this composite is recyclable under the normal catalytic conditions.A prominent researcher Y. Goto et. al. processed a TiO2/CoOOH/RhCrOx/SrTiO3:Al photocatalyst into a 5.0 *5.0 cm sheets & the sustainability of the substance was tested by a panel-type photo reactor with water depth 1.5 mm by simulated light and ambient pressure 117. The water splitting rate of these sheets are 5.7 μmolh−1g−1 and H2 generation efficiency is 0.4% at the initial duration of irradiation. Where according to Hao Lyu et. al the AQY of the resulting RhCrOx/SrTiO3: Al at the time of overall water splintering was measured to be 54.0% at 360.00 nm, which consistent with the ancestral work 118.
Table 9: The recent study of Doping on TiO2 & TiO2 based composite photocatalyst
Year |
Photocatalyst |
Dopant
(metal) |
Reactant Solution |
Light Source |
Efficiency
μmolh−1g−1 |
Ref. |
2019 |
SrTiO3 |
Al |
Pure water |
300.0 W, Xe Lamp |
05.70
|
(118)
|
2018 |
PCN-777 |
Pt |
TEOA |
300 W, |
586.00
|
(116)
|
2018 |
RP/TiO2 |
Red-P |
Methanol |
350.0W, mercury Lamp |
276.00
|
(119)
|
2006 |
Sr3Ti2O7 |
NiOx |
Pure water |
Hg lamp,400–450.0 W |
144.00
|
(120)
|
2002 |
CaTiO3 |
NiOx |
Pure water |
Xe lamp,400–450.0 W |
30.00
|
(121)
|
2002 |
Sr4Ti3O10 |
NiOx |
Pure water |
Xe lamp,400–450.0 W |
170.00
|
(122)
|
1998 |
B/Ti oxide |
Pt |
Pure water |
Hg lamp,400–450.0 W |
22.00
|
(123)
|
1997 |
K2La2Ti3O10 |
NiOx |
Pure water |
Hg lamp,400–450.0 W |
2186.00
|
(124)
|
1997 |
TiO2 |
Pt |
Pure water |
Xe lamp,400–450.0 W |
568.00
|
(125)
|
1995 |
TiO2 |
Pt |
Pure water |
Hg lamp,400–450.0 W |
106.00
|
(126)
|
1987 |
TiO2 |
NiOx |
Pure water |
Hg lamp,400–450.0 W |
62.00
|
(127)
|
1985 |
TiO2 |
Rh |
Pure water |
Xe Lamp, 400–450.0 W |
449.00
|
(128)
|
1980 |
SrTiO3 |
Rh |
Pure water |
Hg lamp, 400–450.0 W |
27.00
|
(129, 130) |
1980 |
SrTiO3 |
NiOx |
Pure water |
Hg lamp, 400–450.0 W |
40.00
|
(127, 131) |
Doping (Copper) on MoS2/Bi2S3 Photocatalyst
In a study, W. P. Cathie Lee fabricated a flower-like Copper-doped molybdenum disulfide/bismuth sulfide (Cu-MoS2/Bi2S3) photo-catalyst. The sample photo-catalysts with differing quantity of Cu were utilized in the photo-catalytic water splitting for H2 production under simulated sunlight irradiation. The photo-catalysts demonstrated high Photocatalytic activity with a most favorable Cu loading of 20.0mol%, reaching a total hydrogen evolution of 32.4 mmol/h on reaction for 6 hours(Fig:-5.0). The Copper doped samples showed increased performance rising about 1.4 times of non-doped samples. The reaction time taken by the different samples are also shown in (Fig: 5-2) respectively 132.
Figure 5: Variation of Hydrogen production rate from variable Cu doping on Na2S/Na2SO3 mixture illuminated under simulated sunlight over the duration of 6.0 h
The crystal structure and surface morphology info of synthesized photo-catalyst were inspected by W. P. Cathie Lee using TEM and FE-SEM 132. The standard structure of non-doped MoS2/Bi2S3 is demonstrated in Fig. 2(a), where MoS2 have been seen to cover surface of Bi2S3, growing on it 132. In each of six images, crumpled MoS2 nano sheets is found to accumulate onto Bi2S3 surface, producing a flower-type frame 134. Upon copper (Cu) doping MoS2/Bi2S3, no notable alteration on samples surfaces were noticed (Fig. 2(b) & (c)). Still, at greater Copper loading to 20.0mol%, on the surface the grown MoS2 sheets were larger enough to be visible and distinguishable (Fig. 2(d)) 132. But on increasing Copper(Cu) to 40.0mol%, the distinct Bi2S3discoid’s not formed, rather some Bi2S3 noticed to be in rod form (indicated as yellow in Fig. 2(f)) where MoS2 sheets were in a arbitrary form 132.
Figure 6: Some images from FE-SEM of MoS2/Bi2S3, (a) Undoped (b) 5.0mol% (c) 10.0mol% (d) 20.0mol% (e) 30.0mol% (f) 40.0mol% 132.
Doping on miscellaneous composite photo-catalyst
Deposition of a foreign particle onto the semiconductor surface can supply maximum active surface for excellent Photo-catalytic H2production. There are many more option with semiconducting properties for Photocatalytic watersplitting. Among them K2La2Ti3O10, possesses a perovskite structure is one of a unique catalyst photocatalysis 135. H2 evolving happens on NiOx co-catalyst where O2 evolves from hydrated interlayer. Various niobate, titanate and tantalite photo-catalysts with perovskite structure been reported from the period of K2La2Ti3O10 photocatalyst was designed. Sr3Ti2O7 and Sr4Ti3O10 photo-catalysts have perovskite slabs of SrTiO3. La2Ti2O7, La2Ti2O7:Ba, KLaZr0.3Ti0.7O4 and La4CaTi5O17photocatalysts with perovskite structure which give a maximum quantum yield. Na2Ti6O13 and BaTi4O9 with a holo-tube type structure is also a unique photo-catalysts. On the other side Catalyst KTiNbO5 shows activity when it prepared through a polymerizable complex composite system. In a study by S. Ikeda et. al. they found thatK2La2Ti3O10 yield 2186μmol Hydrogen in 0.1M KOH solution.124. They used NiOx as dopant and the irradiated light intensity was 400 to 450 W mercury lamp with quartz cell. On the other hand in the year 2006 get 2390μmol Hydrogen from a quartz cell using K3Ta3B2O12 as a catalyst and Pure water Reactant Solution. Table 10 shows a brief summary of recent study on miscellaneous catalyst for Photocatalytic water splitting process. 136.
Table 10: Some study of doping on miscellaneous composite photo-catalyst.
Year |
Photocatalyst |
Dopant
(metal) |
Reactant Solution |
Light Source |
Efficiency
μmolh−1g−1 |
Ref. |
2008
|
LiCa2Ta3O10 |
NiOx |
Pure water |
Hg–Q lamp |
59.00 |
(136) |
2007
|
CeO2:Sr |
RuO2 |
Pure water |
Hg–Q lamp |
110 |
(122) |
2006
|
Na2Ta2O6 |
NiO |
Pure water |
Hg–Q lamp |
391 |
(137) |
2006
|
K3Ta3B2O12 |
None |
Pure water |
Hg–Q lamp |
2390 |
(138) |
2005
|
Cs2Nb4O11 |
NiOx |
Pure water |
Hg lamp, 400–450 W |
1700 |
(139) |
2005
|
NaCa2Nb3O10 |
RuO2 |
Pure water |
Hg–Q lamp |
118 |
(140) |
2005
|
Sr5Ta4O15 |
NiO |
Pure water |
Hg–Q |
1194 |
(141) |
2005
|
Ba5Ta4O15 |
NiO |
Pure water |
Hg–Q |
2080 |
(120) |
2004
|
Y2Ti2O7 |
NiOx |
Pure water |
Hg lamp |
850 |
(142-144) |
2004
|
La3NbO7 |
NiOx |
Pure water |
Hg lamp, 400–450 W |
35 |
(142) |
2003
|
KaLaZr0.3Ti0.7O4 |
NiOx |
Pure water |
Hg lamp, 400–450 W |
230 |
(145) |
2000
|
K2PrTa5O15 |
NiO |
Pure water |
Hg–Q |
1550 |
(146) |
1997
|
Rb2La2Ti3O10 |
NiOx |
0.1 M RbOH |
Hg–Q |
869 |
(147) |
1997
|
K2La2Ti3O10 |
NiOx |
0.1M KOH |
Hg lamp, 400–450 W |
2186 |
(147) |
1996
|
KTaO3 |
Ni |
Pure water |
Hg–Q |
60 |
(148, 149) |
Heterojunction
The heterojunction is the interface that takes place between two layers or sectors of varying crystalline semiconductors. These semiconducting materials have a different band gaps as contrary to a homo junction. Many reviews have shown that various semiconductors can be coupled with BiVO4 for favorable result. The formation of successful hetero junction has been reported between BiVO4 and WO3 150-152 , SnO2 153, 154, Fe2O3 155, 156, CuWO4 157, and CdS 158, 159. Kim et al.showed that hetero type dual photo-anodes, HDP, of BiVO4& a-αFe2O3. 156. Reports also implies largely increased photocurrent on HDP of BiVO4/αFe2O3 becoming permanent. 7 mAcm−2 photocurrent at 1.23V vs. RHE has been reported below 1 solar radiation making subsequent hydrogen production for BiVO4/αFe2O3 nearly 80μmolcm−2h−1 by Savioet et al. On the other hand, the Fe2O3/BiVO4 photo-anode containing 3 spin coated films demonstrated 1.63mAcm−2 photocurrent density at 1.23V vs. RHE, reported by Xia et al. that is nearly 2.2 times higher in comparison to pure BiVO4 photo-anode, submerged in 0.1M KH2PO4 (pH 7) electrolytic solution beneath AM1.5G sun light 155. Rates of hydrogen production for Fe2O3/BiVO4 has been around 28μmolcm−2h−1, quite larger in comparison to BiVO4 photo-anode. A BiVO4/CuWO4 heterojunction electrodes made by Pilli et al. via spray accumulation method was reported two times photocurrent density to pristine BVO at 1.0V vs. Ag/AgCl in 1.0M Na2SO4 electrolytic solution at pH 7. 157. In contrary to pristine BVO, BiVO4/WO3 largely enhanced the IPCE, Incidence of Photon to Current Efficiency, from 9.3% to 31% as reported by Grimes et al. referred it to the phenomenon of enhanced electron migration towards WO3 from BiVO4 160. Also, as reported by Savio et al., BiVO4 and ZnO pairing generate a significant, 2 mA/cm2 at 1.23V vs. RHE, photocurrent density in observable radiation range. 161. Similarly, four times amplified photo current of BiVO4/TiO2, pairing of BiVO4 and TiO2, has been reported by Kimura et al., contrary to pristine BiVO4 on 100mWcm−2 brightness , and they ascribed it to the formational alterations of BiVO4 which improved electron transferring throughout the junction 162.
Dye-Sensitization
For HOMO and LUMO states being reconfigurable on anchorage of various ligands, dye sensitization is considered an effective way to improve the visible range response of photocatalyst for hydrogen generation. Broad usage for dye sensitization is to set visible light to transform energy. Some of the dyes are possible to be utilized in solar cells and Photo-catalytic methods, which have redox property and which are sensitive to visible light 163-165. On illumination of visible light, electrons from excited dyes can be infused to the conduction band of semiconductors, causing inauguration of photo catalytic actions as described in Fig:- 7. Some dyes such as safranine O/EDTA, T/EDTA, are susceptible to take in visible light to generate reducing agents electrons which have enough strength to generate H2 even without semiconductors 166. But it is very little H2 generation rate by dyes without semiconductors 164. Dhanalakshmi et al. carried out a parametric research to study the effect of applying 2þ as a dye sensitizer on Photocatalytic H2 generation from H2O, under visible light irradiation. 167.
Figure 7: Schematic representation of dye sensitizer action mechanism.
Dhanalakshmi et al. observed improved H2 generation rate under visible range radiation due to adsorption of dye molecules to the TiO2 on parametric research studying the influence of applying 2þ as a dye sensitizer on Photocatalyst based H2 generation from H2O. Splitting process 163 . Gurunathan et al. noticed influence of various dyes, for example, EDTA, during H2 generation for SnO2 photocatalyst using or not using sacrificial agents. Having band gap 3.5 eV and, SnO2 was unable to be activated on visible irradiation 168. On dye sensitization, SnO2 was capable of H2 generation on visible range irradiation. As observed, overall order of efficiency for dyes for improving rate of H2 generation is as following: Eosin blue>Rose Bengal>Ru(bpy) 32+>RhodamineB>Acriflavin>Fluorescein 167. By analyzing the phusico-chemical properties ot these dyes, a effective conclusion could be dawn [527]. Like as Rhodamine pose a longer absorption range maxima including more negative potential (.545V) than conduction range (.34V) of SnO2 167. But the problem is that it does not improve the H2 production rate significantly.
Table 11: Absorption wavelength maxima λmax(nm) dyes 169
Sl. No. |
Dye |
Class |
λmax(nm) |
01 |
Thionine (TH+) |
Thiazin |
596 |
02 |
Toluidine blue |
Hiazin |
630 |
03 |
Methylene blue (MB) |
Thiazin |
665 |
04 |
Eosin |
Xanthen |
514 |
05 |
Azure B |
Thiazin |
647 |
06 |
Azure A |
Xanthen |
535 |
07 |
Azure C |
Thiazin |
620 |
08 |
Phenosafranin (PSF) |
Phenazin |
520 |
09 |
Saf-O/SO |
Phenazin |
521 |
10 |
Saf-T/ST |
Phenazin |
524 |
11 |
Neutral red (NR) |
Phenazin |
534 |
12 |
Neutral red (NR) |
Phenazin |
534 |
13 |
Fluorescein |
Xanthen |
490 |
14 |
Erythrosin B |
Xanthen |
525 |
15 |
Dye |
Xanthen |
530 |
Sensitization with Noble metal
Noble or precious metals, comprising Pt, Pd, Au, Ni, Rh, Cu & Ag, proved very efficient to improve photocatalytic activity of TiO2 170, 171. For these noble metals, Fermi levels are lesser in comparison to TiO2 and another similar photocatalyst. The photogenerated electrons transfers towards metal particles from conducting band of photocatalyst, depositing, while holes stay on the Valance Band of the photocatalyst. This decreases the possible rebinding of electron and hole greatly, leading to effective separation and stronger photo-catalytic reaction 172. The prominent researcher Anpo & Takeuchi accustomed ESR, signals to observe transferring of an electron from photo-catalyst towards Pt. Increased Ti3+ signals were observed on increasing irradiation duration and Pt loading decreased the of Ti3+ quantity. This investigation shows electron shifting towards Pt particles from TiO2 (Titanium dioxide). With electron deposition on particles, Fermi levels of the noble metals switch closer to the Conduction Band within TiO2 photo-catalyst leading energy levels towards more negative(-ve) direction which is effectual for splitting water, producing H2 173-175. Small size metal particle accumulation cause huge shifting of Fermi level on negative (-ve) direction on surface of TiO2 catalyst 173. Electrons deposited on the metal particles can move towards H+ upon the surface, adsorbed, and subsequently reduce H+ to form H2. Hence, noble metals having satisfactory work function able to assist in transferring electron, resulting in increased photo-catalytic activity 176. Bamwenda et al. compared H2 generation from water-ethanol solution using TiO2 photo-catalyst loaded with two different metals, Au and Pt. they also examined variant methods for depositing metal particle, for example, deposition–precipitation, photo deposition & impregnation method. 167.
They observed that Pt loaded TiO2 better operated than Au loaded TiO2. On the other hand, Au loading arranged by photo deposition operated better among other methods. Changing could be attributed to improved contact in the photo deposition system between TiO2 active sites and metal particles. But, the preparation system was hardly influencing in case of Pt-loaded TiO2 177. Among others Sakthivel et al. observed good loading in experiment for photo oxidation of acid green 16 treatment with TiO2 photocatalyst loaded with Pt, Au & Pd. As written above, photon absorption by TiO2 can be hampered by too much deposition of metal particles and also it may lead to formation of centers for charge recombination, resulting in less efficiency 177.
Increasing Photo catalytically Active Area
Photo catalytically active field in a photocatalyst is a necessary factor that readily impacts H2 generation rates. So, it was a concern to apply methods for enhancing photo catalytically active area. Two ordinary but efficient ways exist for enhancing the specific surface area. The first way is building uniform nanoparticle-containing thick surfaces and incorporating second material (nanowires, nano cells, nanoparticles, etc.) covering primary photocatalyst exterior is the second way. CdS nanorods as well as nanowire highly active photocatalyst for hydrogen generation can be produced through solvothermal & hydrothermal methods. Also, ultrasonic mediating precipitation, two-step aqueous route, and some additional ways can be effectively used to synthesis mesoporous (CdS) Nanoparticle 44.
Modification of Surface with graphene and other carbonaceous material
At present, Surface moderation of the photo-catalyst is performed with carbonaceous materials such as graphene, graphene quantum dot (GQD)s, carbon nano dots, carbon nanotubes (CNTs) and fullerenes (C60) for improving H2 generation in UV, UV-VM, and visible range area has drawn more remark because of the expanded visible-light absorption range and enhanced charge transfer 44. Graphene exhibits excellent electron mobility (200,000 cm2 V-1 s-1), substantial surface area specific (2630 m2 g-1), superior conductivity, electrical & thermal, and good chemical and physical stableness. Also, it is handily achievable from graphite bulk through mechanical, chemical, and thermal ways 178, 179. Zhang et. al. pointed out the synthesis of grapheme /TiO2Nano composites and the improved photo catalytic hydrogen production through splitting of water, as well as observed that the photo catalytic performance of grapheme /TiO2 Nano composites rely on rGO ratio and calcination process 180. In another work of Li et al., graphene quantum dot(GQD)/ TiO2 composite co-doped with sulfur and nitrogen (S.N-GQD/TiO2 photocatalyst) showed much improved performance than pristine TiO2 caused by improved visible radiation absorbance and efficient dissolution and transferring of photo generated charge 181. Fan et al. systematically conducted an inspection on reduction method influence on the hydrogen production performance of the Nano composite TiO2/RGO, and observed that Nano composite produced through hydrothermal method showed the best performance in H2 generation activity on UV-Vis light radiation 182 . Also, Fan el al. observed that grapheme quantum dot anchored TiO2 showed improved photo catalytic hydrogen generation performance on using methanol solution because of graphene quantum dots(GQD) operating as effective reservoirs for electron and fantastic photo sensitizer’s for Titanium dioxide 183.
Formation of Solid Solutions
A Solid solution production for compressing band-gap of semiconductors is a proper way to enhance efficiency for visible light absorption and utilization. Solid solution of ZnS and AgInS2, (AgIn) xZn2(1-x)S2 consisting of a compressed band-gap exhibited high visible-light photo-catalytic hydrogen production activities from aqueous S2- and SO32- solutions for sacrificial purpose.On AgInS2 ratio increment to ZnS, the light absorbance of (AgIn) xZn2(1-x)S2 solid solutions transferred to larger wavelengths because the photo-physical, photo-catalytic activities of (AgIn) xZn2(1-x)S2 solid solutions were dependent upon the structure changing for band position variation 44. In addition, easily produced solid solutions from same crystal formation ZnS and CdS, ZnS-CdS photocatalyst are fantastically responsive towards visible-light for hydrogen generation 184.
Reactor Design & Development
A chemical reactor is an enclosed system in which chemical changes are takes place. The scheme/model of a chemical reactor deals with multiplex aspects of chemical engineering. Chemical engineers design a reactor to maximize the efficiency for the given reaction. Reactor modeling is a significant part in photo-catalytic water splitting systems as well as hydrogen production plants.
Factors to be consider for reactor design:
Geometrical set-up of the reactor
Source of radiation (Natural or artificial)
Catalyst (Slurry or hold in support)
Sacrificial agents (CH3OH, Waste Water, etc.)
Light source position (35 – 450 angle, Concentric)
Sacrificial agents
Irradiation time
Shelf-life
Photo-reactor Design
The photo-reactor is any chamber or vessel where reaction between the photo-catalyst and reactants takes place with the presence of photon (light) and Sacrificial reagents (where needed). Different types of reactor based on UV and visible light irradiation has been shown in Fig: 8 28. The photo-catalyst and light source are the main constituents of photo-reactor. For better photo-catalytic performance, an ideal photo-reactor must have uniform light distribution. 185. Tactics of operation and phase are important factors which determine the types of photo-reactor. Here Table-12 demonstrates the benefits and drawbacks of different photo-reactors. Table-12 summarized the benefits and drawbacks of different types of photo-reactor.
Figure 8: Types of photo-reactors for visible and UV light photo-catalytic to produce hydrogen 28.
Batch-type photo reactor
The batch type reactor is the primary photo reactor in reactor types. Here Fig: 9.0 exhibits a batch type photo reactor for the catalytic hydrogen evolution from water. This types of the batch reactor are consist of a catalysts bed, reaction vessel, cooling water system, Quartz windows, light source, evacuation system, sample collection system, and gas detection device 28, 186. The water jacket around the reactor circulates water continuously to control the reactor and reaction temperature at a particular range. Light source will emit rays along the quartz windows.
Figure 9: Pictorial representation of a typical photo reactor built with stainless steel vessel and quartz oxeye 28.
Continuous annular photo-reactors
Continuous annular photo-reactors are composed of a ring shaped reactor with a light source located in the center. The inert N2 environment is maintained Into the reactor, to isolate the system from the surroundings. A constant flow of Ar gas bubble is provided through the reaction slurry to keep the suspension homogeneous. To analyze, a portion of the produced hydrogen gas is inserted to a gas valve of gas chromatography (GC). The benefit of continuous annular photo-reactors is to enhance the liquid-gas interfacial area, which simplifies the release of H2 from the photo-catalyst surface. One of the major drawbacks of these types of the reactor is, more photon can be received by the interior of the annular reactor than that of the exterior.
Photo catalytic membrane reactor
Photo catalytic membrane reactor (PMRs) is effective for sustainable production of hydrogen by suppressing the reversed reaction between O2 and H2. Pure hydrogen can be obtained in a single step without further purification. In the case of PMRs design, photo-catalyst and metal loading, the mechanical resistance, permeability, membrane morphology are important factors to be considered and needed to get excellent efficiency of catalytic membrane system while in hydrogen evolution. There are various types of PMRs for hydrogen evolution, such as H-type Photo-catalytic reactor (HPR), membrane electrode photo-catalyst assembly (MEPA), membrane twin reactor (MTR), and polymer membrane electrode assembly (PMEA) 28.
Optical Fiber and Honeycomb Reactors
Generally Optical fibers, made of silica, are used to transmit light from one end to another end of the fibers. Light is split into two parts by the variation of refractive index between the quartz core and the semiconductor, such as TiO2, coating during hitting the internal surface of the fiber 28. Some portion of the light is transmitted and reflected through the fiber, while the rest fraction excites and penetrates the titanium layer on the interface. Thus, photo-reactions occur through the formation of electron-hole pair. As a result, optical fibers are utilized to radiate the light properly in the interior of a photo-reactor 187. Light is passed through the fibers core by forming a cover which is lower refractive index that captures light beam in the core through total internal reflection 188. Formerly, H2 production rate was increased through photo catalytic water splitting using optical fiber coated with SiO2 and TiO2 189. There are several benefits of optical fibers such as: It has higher efficiency enlighten the interior of reactor uniformly and it causes higher interactions between irradiations and catalyst surface.
Figure 10: The pictorial representation of an optical fiber honeycomb reactor185
Monolith reactor
It is a multifunctional reactor which is integrated with separation and heat exchanger. The designs of a monolith reactor are uniform sets with a parallel line that can be constructed into various configurations (sizes and shapes). Application of monolith reactor having a narrow path with larger light beam interaction surface area (SA) can enhance the transformation and product rates 190. Because of its excellent properties the Ceramic monolithic reactor formations shows an attractive choice to commonly designed catalyst nubbles or powders. Monoliths has several advantages over typical catalyst powders or nubbles, such as: improved weight transfer rate, good coating adhesion, better porosity, minimal pressure fall, better thermal and mechanical strength 191. Current improvement in photo (light) technology exhibited that, when compared with other commercial reactors, the monolith reactors have several benefits over them. In 2017, splitting of water for hydrogen evaluation with sacrificial agent, ethanol, in a monolith photo-reactor has been tested by Gaudillere et al. 192. According to Tahir Met. al., the quantum efficiency (QE) in micro-channel monolith reactor gave much higher (0.10%) than deal with cell type reactor (0.0005%).193 In another analysis performed by Tahir Met. al. they used/applied photo catalyst NiOeIn2O3/TiO2 which showed superior performance in the batch type monolith photo reactor than considering cell type reactor under identical conditions of reaction with efficiency of 14.13 times higher 194.
Particulate photo catalytic reactor
Particulate photo catalyst reactor systems are narrated here having some facilities in case of designing cost and scale-up potential as compared with photo electrochemical and photovoltaic systems 195. However, the development of photo catalytic water splitting set up even to the one to one meter scale has rarely been published, although the ultimate demand of fabricating plants tens of square kilometers 186. Elsewhere, the power generation through photovoltaic system have been installed and commercialized in an extend phase. This indicates a non-stop challenge to the application of photo-catalytic splitting of water as a way of production of solar fuel.
Figure 11: The pictorial representation of a photo-reactor (large scale) for photo-catalytic reactions with an immobilized photo-catalyst.186
Formerly, photo-catalytic water splitting has been studied in flask-type reactors using suspensions of photo-catalyst powders on the small-scale Nevertheless, the disadvantage in the development of suspension systems can be guessed from the deficiency of big-scale displays of water splitting by sunlight overall with noticeable values of STH. Honestly, this an attempt is annoying on big scales for various factors 186. Firstly, it is challenging to lessen the costs of reactor, such as huge water is required. For example, 1 cm depth, water quantity in a reactor increases in values of 10 kg one square meter and reactors seem to be larger and high cost. This is not be feasible on a big scale production process assuming that the highest cost allowed for the complete hydrogen production systems is about US$102 m-2, as mentioned earlier 196. Secondly, there is a tendency of the particulate photo-catalysts to precipitate to the basement of the reactor if not the reactor is positioned perfectly. They do not receive incident light effectively too. Tubular shaped reactors combined with a CPC (compound parabolic concentrator) have been experimented for big scale industrial operation of photo-catalytic hydrogen evolution reactions (HER) in the availability of sacrificial reagents (SR) 197. In the research done by Jing et al., CPCs contain the highest half incident beam angle of 14°, length of 0.4 m, and whole arm of 1.5 m, and pyrex reactor tubes 1.6 m in length were used. However, as particulate photo-catalytic reactor is scalable and it has low operation and maintenance cost, it is more effective than others.
Table 12: The benefits and drawbacks of different types of photo-reactors:
Reactor
|
Findings |
Drawbacks |
Ref. |
Slurry reactor
|
- Can be operated in fixed bed mode or continuous flow patterns
- Combination of gas-liquid-solid phase
- Continuous stirring causes the additional cost
- Active contact surface for reaction is low
|
|
(28, 185)
|
Fluidized reactor
|
- High photo-catalytic activity
- Efficient rate of heat and matter transfer by
vigorous excitation of solid
- Abrasion of particles and attrition of the catalyst may
causes erosion in reactor
- difficult to separate from mixture
|
|
(188, 198)
|
Optical reactor
|
- Surface area is larger
- Efficiency of light utilization is higher
- Efficient processing capacities of the catalyst
- Deactivation catalyst at high temperature
- Maximum reactor volume cannot be applying
Uniform coating of fibers is complex
|
|
(188)
|
Monolith reactor
|
- Ratio of surface to volume is higher
- Pressure drop is low
- Flow rate is higher
- Light efficiency is low
- Catalyst adhesion on wall is lower
|
|
(188, 198, 199)
|
Fixed bed reactor
|
- large Surface area
- minimum reaction period
- lower Operating cost
- low Light efficiency
- lower adhesion
|
|
(188, 198, 199)
|
Particulate photo-catalyst reactor
|
- Minimal fabrication cost
- High scale up potential
- Operating cost is lower
- reactors tend to be bulky
- water is required in large volumes
|
|
(186)
|
Process Parameters for photo catalytic reaction
Light intensity or Irradiation of light
Temperature effect
PH effect
Reactor design
Oxygen vacancies
Presence of Sacrificial agents
Miscellaneous factors affect
Light intensity
The higher hydrogen production rate is linearly related to light irradiation. The effectiveness of photo catalytic splitting of water can be improved with the rise in light intensity generating power more than the threshold level of activation 200,201. Two regions are available related to the photo-catalytic reaction related to the UV photon flux. One region is for laboratory research fluxes which are 25 mW cm-2. Compare to the reaction of recombination the hole electron couple is consumed quicker in a chemical reaction. During the half-order region, the intensity remains higher, the recombination rate is a major factor and creates less change on the rate of the change the reaction process. The change of the value of the reaction rate is related to the wavelength which appears following the catalyst spectrum of the adsorption with a minimum reading level with respect to the band potential energy 202 . In the year 2013, Baniasadi et. alannounced that the rate of hydrogen generation from Zinc-Sulfide (ZnS), increased by 20% with rise in intensity range of light from 90 to 100 mW cm-2 203. In another research work report by Leon and Tambago, indicated that the hydrogen (H2) production increased using Cd0.4Zn0.6S while the intensity of light was increased 204.
Figure 12: The comparison ofphoto-catalysts for hydrogen (H2) generationunder irradiation of solar Light for TNS(titanium nano sheet), TNT(titanium nano tube) and TNR(titanium nano rod)205
Temperature effect
The photo-catalytic behavior of catalyst material is introduced by temperature, since electron and hole generation is not related to it directly. But, the temperature has a crucial role to improve desorption of hydrogen gas (H2) from the surface area of the considering catalyst to accelerate the effect of photo catalyst. It is thermodynamically established that, temperature ameliorates the reaction process in maximum cases. The rate of change of applied temperature differs according to the change of catalyst. The photo-catalytic activity could be increased by the adjustment of the factor. The hydrogen (H2) production becomes slower when the value of the temperature goes down. In this process desorption limits the reaction mechanism. The higher valance electron in the valance band will be transferred to higher energy levels during high-temperature conditions. The electron and hole generation will initiate oxidation & reduction reaction respectively with charge carrier recombination 202. In 30℃, 40℃, 50℃ the hydrogen gas generation was 59, 92 & 370 mol/g.s respectively 206. Hydrogen generation increased from 4.71 mmol g1 to 15.18 mmol g1 after introducing a temperature rise from 45℃ to 55℃207. In a photocatalytic study it was found that the optimum temperature of this process should be 60℃ to 80℃.208
Table 13: Temperature effect on H2 production rate.
Sl. No. |
Temperatuire0C |
H2 production rate |
Catalyst |
01 |
30 0C |
59.00 mol/g.s |
Pt/TiO2 |
02 |
40 0C |
92.00 mol/g.s |
Pt/TiO2 |
03 |
50 0C |
370.00 mol/g.s |
Pt/TiO2 |
pH Effect
The hydrogen (H2) generation from water splitting depends on the concentration of the proton which indicates the pH of the regarding solution. In the water-splitting process, an electron is generated throughout the reaction process. A significant organic species is needed for the proton reforming. It is recommended to generate hydrogen in a strongly acidic solution rather than in a basic solution (pH>7). Similarly, the band gap potential shift also depends on pH value [20]. In a CuOx/TiO2 catalytic medium with basic medium (pH 10) hydrogen production is maximum. The atom copper Cu (I) shows unstable behavior in TiO2 based acidic medium. In the visible light TiO2 part of the catalyst, Si/CdS/TiO2/Pt is unstable in a strong base or acidic reaction 209. The optimum pH value of a photo-catalytic reaction for better hydrogen production rate in CuAlO2/TiO2 catalyst surface is 11 210. The catalyst Pt/r-TiO2 has the ability to produce hydrogen up to 56.6mmol in a pH condition at 5.5 during 4h of operation from pH value 12 to 2.0 211. The hydrogen generation is 1200mmol g-1 h-1 in NiO/TiO2 catalyst in a pH condition 6.6.So the hydrogen generation is directly depends on the according pH reading of the reaction where the best pH reading is about zero [0.00] charge value 212.In an acidic solution of methanol-water the photosensitized TiO2/RuO2-MV2+ catalyst can generate enough hydrogen (H2) 213. The reduction reaction of hydrogen from H+ to H2 can be increased when more protons are present on the surface of the regarding catalyst in an acidic solution (pH<7).The hydrogen generation is rapid in the acidic solution than in the basic solution 203. So in a photo-catalytic reaction the hydrogen production is better in the acidic solution.
Oxygen vacancy
Oxygen vacancy is a significant factor to enhance H2 production as well as the ionic longevity increase. Paucity of oxygen can be produced by applying metal oxides with the process of catalyst synthesis, decreasing value and mixture 214. When the titanium-oxide (TiO2) is used as the titanium ion and oxygen gap are created at the same time. More titanium ions are produced because of the increasing oxygen gap. As a result, there is a situation of titanium defects, amorphous surface area disorder, and finally relative oxygen gap. This oxygen gap indirectly related to electron stability because of the absence of oxygen ion. Whereas, the regular lattice of the oxygen atom was taken by electrons, and the local state was formed by oxygen vacancies and Ti3+. Then, the VB holes of TiO2 were generated and the electrons excited to the CB of TiO2. At CB, the H+ reduce to H2 through a reduction reaction 215. So hydrogen gas generation without the recombination of the electron-hole and the amelioration of the charge longevity is the main aim to be achieved.
Reagent for Sacrificial behavior
By introducing organic species in the reaction process the efficiency of the photo-catalyst can be improved. For example Methanol, Ethanol, phenol, and Glycerol can act as reaction agents which will be sacrificial. Relative to the water molecule, organic species behaves like a hole which will be a scavenger 216. Photo-catalysis process is one of the major way of sustainable hydrogen production. Now the effect of the sacrificial agents in the way of hydrogen production must be fruitful. This hydrogen production can be accelerated using alcohol. This process is also known as photo-reforming. Because alcohol helps semiconductor in oxidation and reduction reaction. The activity order of sacrificial reagents is, Glycerol > Ethylene glycol > Methanol > Ethanol 217. The hydrogen gas generation is a dependable factor with respect to the alpha hydrogen. Glycerol has a unique structure with five alpha hydrogen which ismajor than glycol, methanol and ethanol. On the other hand hydrogen generation is also have a relationwith the concentration of alpha hydrogen and concentration of the agents. 218
Figure 13: The photo-catalysts comparison for hydrogen generation in the irradiation of solar Light for TNS, TNT and TNR 205.
Figure:13 exhibits the outcome rate of hydrogen with the increment of methanol percentage and reaction time. It shows that at5%methanol concentration the value hydrogen production rate is optimum. But further concentration rise does not help further in hydrogen production. The reason behind it may be the saturation of catalyst surface. When the surface area of the photo-catalyst goes to a saturated level then the hydrogen production does not rise further. So only a certain level of hydrogen production rate can be maintain from all the arrangement.
Table 16:A Summary of numerous sacrificial reagents used in photo-catalytic H2 production process.
Year |
Feed |
Catalyst |
Reactor/Parameter |
Efficiency |
Ref. |
2019 |
10 % ethanol-water |
.05 g TiO2/MMT
Quartz flask |
Slurry ractor
40 W Xe lamp |
methanol >ethylene
glycol > ethanol >>propanol >Glycerol |
(219) |
2017 |
15% glycerol-water
|
85 mg
0.5 wt% Pt//TiO2 |
Pyrex reactor
130 W Hg lamp
Medium pressure |
Pt >Pd> Au
glycerol > Propan-2-ol |
(220) |
2016 |
25 % n-propanol -water
|
50 mg 0.5 %RuO2@TiO2 |
Glass reactor
UV light
Temperature at 25 C |
MeOH> t-BuOH > n-BuOH, PrOH> n-EtOH |
(221) |
2015 |
15% 2-propanol -water
|
0.065 g &1 wt% Pd
|
Tubular reactor
UV (375 nm, 10 mW cm-2) |
Pd> Pt z Au.
methanol > 2- ethanol
>propanol >tert-butanol |
(222) |
2015 |
5% methanol-water |
450 mL water solution,
|
Quartz reactor system
250 W Xe arc lamp,
30 C under atmospheric pressure |
ethylene > anhydrous glycol >Methanol
|
(223) |
2015 |
5 % glycol-water |
6.5 mg 1.5 wt% Au/P25
|
Tubular reactor
SB-100P/F lamp (100 W,
265 nm) |
Au/P25 > Au/brookite> Au/anatase |
(217) |
Conclusions
In comparison with cleanness and high calorific value Hydrogen is now a burning issue for today’s energy regulatory authorities rather than other fuels like fossil, wind, solar, or another renewable. However, in nature H2 is not available in free form (i.e. atomic state). Among various H2 production process low-cost H2 is available from fossil i.e. petrochemicals, but the main question that arises here is the sustainability of this process. To ensure affordable and clean energy (SDG Goal-7) Sunlight to Hydrogen or STH is the best solution. While comparing with other traditional fuels H2 is a Future dominating fuel with its best transportation, storage, transmission, and cleanness. Photo catalysis is the best way to achieve maximum STH conversion with less set-up and reagent cost. However, there are still so many technical drawbacks in catalytic water splitting as this technique still not mature enough. Several researchers are trying to develop this process from the different technical corners like catalyst development, process parameter optimization, reactor design, etc. Some other catalyst development techniques like Sensitization with noble metal loading, Increasing Photo catalytically Active Area, modification of Surface with graphene and other Carbonaceous material, Formation of Solid Solutions, and Modification of Photo-catalysts with Co-catalysts are also studied to develop Photocatalytic efficiency of catalysts. Among all techniques doping, Heterojunction and Dye sensitization shed a light on development techniques for efficient catalyst synthesis. Within this three-technique, the development of doping in the different catalysts is the most promising one. Doping in the catalyst surface may extend its photonic response from the UV region to the visible length of electromagnetic radiation by forming an impure energy band. However, there is still a lot to work on the photocatalytic water splitting process for better understanding and smooth future of this process.
Highlights
Photo-catalytic water splitting is a promising Green-Harvesting technique among all H2 generation methods
Doping, Heterojunction and Dye sensitization shed a light on development strategies
Elemental doping exhibit’s maximum efficiency while doping with rare earth metals
Acknowledgement
The authors would like to acknowledge Bangladesh Council of Scientific and Industrial Research (BCSIR) &Hydrogen Energy Laboratory project for its Scholastic and technical support.
Conflict of Interests
The author(s) declared no potential competing interest with respect to the research, authorship, and/or publication of this article.
Funding Sourse
This research did not receive any specific grant from funding agencies in the public or commercial sectors.
References
- Shafiee S, Topal E. When will fossil fuel reserves be diminished? Energy policy. 2009;37(1):181-9.
CrossRef
- Höök M, Tang X. Depletion of fossil fuels and anthropogenic climate change—A review. Energy policy. 2013;52:797-809.
CrossRef
- Bhandari R, Trudewind CA, Zapp P. Life cycle assessment of hydrogen production via electrolysis–a review. Journal of cleaner production. 2014;85:151-63.
CrossRef
- Kumaravel V, Mathew S, Bartlett J, Pillai SC. Photocatalytic hydrogen production using metal doped TiO2: A review of recent advances. Applied Catalysis B: Environmental. 2019;244:1021-64.
CrossRef
- Balta MT, Dincer I, Hepbasli A. Thermodynamic assessment of geothermal energy use in hydrogen production. International Journal of hydrogen energy. 2009;34(7):2925-39.
CrossRef
- Dincer I, Acar C. Review and evaluation of hydrogen production methods for better sustainability. International journal of hydrogen energy. 2015;40(34):11094-111.
CrossRef
- Dincer I. Green methods for hydrogen production. International journal of hydrogen energy. 2012;37(2):1954-71.
CrossRef
- Acar C, Dincer I, Naterer GF. Review of photocatalytic water‐splitting methods for sustainable hydrogen production. International Journal of Energy Research. 2016;40(11):1449-73.
CrossRef
- Kotay SM, Das D. Biohydrogen as a renewable energy resource—prospects and potentials. International Journal of Hydrogen Energy. 2008;33(1):258-63.
CrossRef
- Koutrouli EC, Kalfas H, Gavala HN, Skiadas IV, Stamatelatou K, Lyberatos G. Hydrogen and methane production through two-stage mesophilic anaerobic digestion of olive pulp. Bioresource technology. 2009;100(15):3718-23.
CrossRef
- Das D, Veziroglu TN. Advances in biological hydrogen production processes. International journal of hydrogen energy. 2008;33(21):6046-57.
CrossRef
- Lodhi M. Hydrogen production from renewable sources of energy. International journal of hydrogen energy. 1987;12(7):461-8.
CrossRef
- Guo L, Chen Y, Su J, Liu M, Liu Y. Obstacles of solar-powered photocatalytic water splitting for hydrogen production: A perspective from energy flow and mass flow. Energy. 2019;172:1079-86.
CrossRef
- Fujishima A, Honda K. Electrochemical photolysis of water at a semiconductor electrode. nature. 1972;238(5358):37-8.
CrossRef
- Zou Z, Ye J, Sayama K, Arakawa H. Direct splitting of water under visible light irradiation with an oxide semiconductor photocatalyst. Materials For Sustainable Energy: A Collection of Peer-Reviewed Research and Review Articles from Nature Publishing Group: World Scientific; 2011. p. 293-5.
CrossRef
- Wang H, Zhang L, Chen Z, Hu J, Li S, Wang Z, et al. Semiconductor heterojunction photocatalysts: design, construction, and photocatalytic performances. Chemical Society Reviews. 2014;43(15):5234-44.
CrossRef
- Chen X, Shen S, Guo L, Mao SS. Semiconductor-based photocatalytic hydrogen generation. Chemical reviews. 2010;110(11):6503-70.
CrossRef
- An X, Hu C, Liu H, Qu J. Hierarchical nanotubular anatase/rutile/TiO2 (B) heterophase junction with oxygen vacancies for enhanced photocatalytic H2 production. Langmuir. 2018;34(5):1883-9.
CrossRef
- Zhang C, Zhou Y, Bao J, Zhang Y, Fang J, Zhao S, et al. Sn2+-Doped Double-Shelled TiO2 Hollow Nanospheres with Minimal Pt Content for Significantly Enhanced Solar H2 Production. ACS Sustainable Chemistry & Engineering. 2018;6(5):7128-37.
CrossRef
- Hainer AS, Hodgins JS, Sandre V, Vallieres M, Lanterna AE, Scaiano JC. Photocatalytic hydrogen generation using metal-decorated TiO2: sacrificial donors vs true water splitting. ACS Energy Letters. 2018;3(3):542-5.
CrossRef
- Xu Q, Cheng B, Yu J, Liu G. Making co-condensed amorphous carbon/g-C3N4 composites with improved visible-light photocatalytic H2-production performance using Pt as cocatalyst. Carbon. 2017;118:241-9.
CrossRef
- Jo W-K, Selvam NCS. Z-scheme CdS/g-C3N4 composites with RGO as an electron mediator for efficient photocatalytic H2 production and pollutant degradation. Chemical Engineering Journal. 2017;317:913-24.
CrossRef
- Liu X, Wang P, Zhai H, Zhang Q, Huang B, Wang Z, et al. Synthesis of synergetic phosphorus and cyano groups (CN) modified g-C3N4 for enhanced photocatalytic H2 production and CO2 reduction under visible light irradiation. Applied Catalysis B: Environmental. 2018;232:521-30.
CrossRef
- Vaquero F, Navarro R, Fierro J. Influence of the solvent on the structure, morphology and performance for H2 evolution of CdS photocatalysts prepared by solvothermal method. Applied Catalysis B: Environmental. 2017;203:753-67.
CrossRef
- Zhang M, Lin H, Cao J, Guo X, Chen S. Construction of novel S/CdS type II heterojunction for photocatalytic H2 production under visible light: the intrinsic positive role of elementary α-S. Chemical Engineering Journal. 2017;321:484-94.
CrossRef
- Qin N, Xiong J, Liang R, Liu Y, Zhang S, Li Y, et al. Highly efficient photocatalytic H2 evolution over MoS2/CdS-TiO2 nanofibers prepared by an electrospinning mediated photodeposition method. Applied Catalysis B: Environmental. 2017;202:374-80.
CrossRef
- Dincer I, Acar C. A review on clean energy solutions for better sustainability. International Journal of Energy Research. 2015;39(5):585-606.
CrossRef
- Fajrina N, Tahir M. A critical review in strategies to improve photocatalytic water splitting towards hydrogen production. International Journal of Hydrogen Energy. 2019;44(2):540-77.
CrossRef
- Haselmann GM, Eder D. Early-stage deactivation of platinum-loaded TiO2 using in situ photodeposition during photocatalytic hydrogen evolution. ACS Catalysis. 2017;7(7):4668-75.
CrossRef
- Tiwari A, Krishna NV, Giribabu L, Pal U. Hierarchical porous TiO2 embedded unsymmetrical zinc–Phthalocyanine sensitizer for visible-light-Induced photocatalytic H2 production. The Journal of Physical Chemistry C. 2018;122(1):495-502.
CrossRef
- Guayaquil-Sosa JF. Photocatalytic Hydrogen Production using a Mesoporous TiO2 Doped with Pt: Semiconductor Synthesis, Oxidation-Reduction Network and Quantum Efficiencies. 2018.
CrossRef
- Hou H-J, Zhang X-H, Huang D-K, Ding X, Wang S-Y, Yang X-L, et al. Conjugated microporous poly (benzothiadiazole)/TiO2 heterojunction for visible-light-driven H2 production and pollutant removal. Applied Catalysis B: Environmental. 2017;203:563-71.
CrossRef
- Seadira TW, Sadanandam G, Ntho T, Masuku CM, Scurrell MS. Preparation and characterization of metals supported on nanostructured TiO2 hollow spheres for production of hydrogen via photocatalytic reforming of glycerol. Applied Catalysis B: Environmental. 2018;222:133-45.
CrossRef
- Murakami N, Chiyoya T, Tsubota T, Ohno T. Switching redox site of photocatalytic reaction on titanium (IV) oxide particles modified with transition-metal ion controlled by irradiation wavelength. Applied Catalysis A: General. 2008;348(1):148-52.
CrossRef
- Gholipour MR, Dinh C-T, Béland F, Do T-O. Nanocomposite heterojunctions as sunlight-driven photocatalysts for hydrogen production from water splitting. Nanoscale. 2015;7(18):8187-208.
CrossRef
- Avilés-García O, Espino-Valencia J, Romero R, Rico-Cerda JL, Arroyo-Albiter M, Natividad R. W and Mo doped TiO2: synthesis, characterization and photocatalytic activity. Fuel. 2017;198:31-41.
CrossRef
- Hussain M, Ceccarelli R, Marchisio D, Fino D, Russo N, Geobaldo F. Synthesis, characterization, and photocatalytic application of novel TiO2 nanoparticles. Chemical Engineering Journal. 2010;157(1):45-51.
CrossRef
- Zhang G, Lin L, Li G, Zhang Y, Savateev A, Zafeiratos S, et al. Ionothermal Synthesis of Triazine–Heptazine‐Based Copolymers with Apparent Quantum Yields of 60% at 420 nm for Solar Hydrogen Production from “Sea Water”. Angewandte Chemie International Edition. 2018;57(30):9372-6.
CrossRef
- Sudha D, Sivakumar P. Review on the photocatalytic activity of various composite catalysts. Chemical Engineering and Processing: Process Intensification. 2015;97:112-33.
CrossRef
- Bashiri R, Mohamed NM, Kait CF, Sufian S. Hydrogen production from water photosplitting using Cu/TiO2 nanoparticles: Effect of hydrolysis rate and reaction medium. International Journal of Hydrogen Energy. 2015;40(18):6021-37.
CrossRef
- Huang H, Dai B, Wang W, Lu C, Kou J, Ni Y, et al. Oriented built-in electric field introduced by surface gradient diffusion doping for enhanced photocatalytic H2 evolution in CdS nanorods. Nano letters. 2017;17(6):3803-8.
CrossRef
- Shi R, Ye HF, Liang F, Wang Z, Li K, Weng Y, et al. Interstitial P‐Doped CdS with Long‐Lived Photogenerated Electrons for Photocatalytic Water Splitting without Sacrificial Agents. Advanced Materials. 2018;30(6):1705941.
CrossRef
- Zhang L, Liu D, Guan J, Chen X, Guo X, Zhao F, et al. Metal-free g-C3N4 photocatalyst by sulfuric acid activation for selective aerobic oxidation of benzyl alcohol under visible light. Materials Research Bulletin. 2014;59:84-92.
CrossRef
- Deng F, Zou J-P, Zhao L-N, Zhou G, Luo X-B, Luo S-L. Nanomaterial-Based Photocatalytic Hydrogen Production. Nanomaterials for the Removal of Pollutants and Resource Reutilization: Elsevier; 2019. p. 59-82.
CrossRef
- Chen M, Liu Y, Li C, Li A, Chang X, Liu W, et al. Spatial control of cocatalysts and elimination of interfacial defects towards efficient and robust CIGS photocathodes for solar water splitting. Energy & Environmental Science. 2018;11(8):2025-34.
CrossRef
- Munoz-Batista MJ, Fontelles-Carceller O, Ferrer M, Fernández-García M, Kubacka A. Disinfection capability of Ag/g-C3N4 composite photocatalysts under UV and visible light illumination. Applied Catalysis B: Environmental. 2016;183:86-95.
CrossRef
- Singh R, Dutta S. A review on H2 production through photocatalytic reactions using TiO2/TiO2-assisted catalysts. Fuel. 2018;220:607-20.
CrossRef
- Chiu I, Lin S-X, Kao C-T, Wu R-J. Promoting hydrogen production by loading PdO and Pt on N–TiO2 under visible light. International journal of hydrogen energy. 2014;39(27):14574-80.
CrossRef
- Lai C-L, Huang H-L, Shen J-H, Wang K-K, Gan D. The formation of anatase TiO2 from TiO nanocrystals in sol–gel process. Ceramics International. 2015;41(3):5041-8.
CrossRef
- Sun T, Liu E, Fan J, Hu X, Wu F, Hou W, et al. High photocatalytic activity of hydrogen production from water over Fe doped and Ag deposited anatase TiO2 catalyst synthesized by solvothermal method. Chemical engineering journal. 2013;228:896-906.
CrossRef
- Tian M, Wang H, Sun D, Peng W, Tao W. Visible light driven nanocrystal anatase TiO2 doped by Ce from sol–gel method and its photoelectrochemical water splitting properties. International journal of hydrogen energy. 2014;39(25):13448-53.
CrossRef
- Gao H, Zhang P, Zhao J, Zhang Y, Hu J, Shao G. Plasmon enhancement on photocatalytic hydrogen production over the Z-scheme photosynthetic heterojunction system. Applied Catalysis B: Environmental. 2017;210:297-305.
CrossRef
- Fan X, Fan J, Hu X, Liu E, Kang L, Tang C, et al. Preparation and characterization of Ag deposited and Fe doped TiO2 nanotube arrays for photocatalytic hydrogen production by water splitting. Ceramics International. 2014;40(10):15907-17.
CrossRef
- Fornari AMD, de Araujo MB, Duarte CB, Machado G, Teixeira SR, Weibel DE. Photocatalytic reforming of aqueous formaldehyde with hydrogen generation over TiO2 nanotubes loaded with Pt or Au nanoparticles. International Journal of Hydrogen Energy. 2016;41(27):11599-607.
CrossRef
- Xing Z, Zong X, Butburee T, Pan J, Bai Y, Wang L. Nanohybrid materials of titania nanosheets and plasmonic gold nanoparticles for effective hydrogen evolution. Applied Catalysis A: General. 2016;521:96-103.
CrossRef
- Zou Y, Kang S-Z, Li X, Qin L, Mu J. TiO2 nanosheets loaded with Cu: A low-cost efficient photocatalytic system for hydrogen evolution from water. International journal of hydrogen energy. 2014;39(28):15403-10.
CrossRef
- Badawy MI, Ali ME, Ghaly MY, El-Missiry MA. Mesoporous simonkolleite–TiO2 nanostructured composite for simultaneous photocatalytic hydrogen production and dye decontamination. Process Safety and Environmental Protection. 2015;94:11-7.
CrossRef
- Tian H, Kang S-Z, Li X, Qin L, Ji M, Mu J. Fabrication of an efficient noble metal-free TiO2-based photocatalytic system using Cu–Ni bimetallic deposit as an active center of H2 evolution from water. Solar Energy Materials and Solar Cells. 2015;134:309-17.
CrossRef
- Yan Z, Yu X, Han A, Xu P, Du P. Noble-metal-free Ni (OH) 2-modified CdS/reduced graphene oxide nanocomposite with enhanced photocatalytic activity for hydrogen production under visible light irradiation. The Journal of Physical Chemistry C. 2014;118(40):22896-903.
CrossRef
- Chiarello GL, Dozzi MV, Scavini M, Grunwaldt J-D, Selli E. One step flame-made fluorinated Pt/TiO2 photocatalysts for hydrogen production. Applied Catalysis B: Environmental. 2014;160:144-51.
CrossRef
- Ong W-J, Tan L-L, Ng YH, Yong S-T, Chai S-P. Graphitic carbon nitride (g-C3N4)-based photocatalysts for artificial photosynthesis and environmental remediation: are we a step closer to achieving sustainability? Chemical reviews. 2016;116(12):7159-329.
CrossRef
- Kang HW, Park SB, Kim JG, Kim IT. Effects of F for enhancement of H2 evolution on visible-light-driven photocatalyst of SrTiO3: Cr/Ta/F prepared by spray pyrolysis. International journal of hydrogen energy. 2014;39(11):5537-45.
CrossRef
- Shen P, Lofaro Jr JC, Woerner WR, White MG, Su D, Orlov A. Photocatalytic activity of hydrogen evolution over Rh doped SrTiO3 prepared by polymerizable complex method. Chemical engineering journal. 2013;223:200-8.
CrossRef
- Zhang H, Chen G, Li Y, Teng Y. Electronic structure and photocatalytic properties of copper-doped CaTiO3. International journal of hydrogen energy. 2010;35(7):2713-6.
CrossRef
- Sun X, Xie Y, Wu F, Chen H, Lv M, Ni S, et al. Photocatalytic hydrogen production over chromium doped layered perovskite Sr2TiO4. Inorganic chemistry. 2015;54(15):7445-53.
CrossRef
- Zou J-P, Zhang L-Z, Luo S-L, Leng L-H, Luo X-B, Zhang M-J, et al. Preparation and photocatalytic activities of two new Zn-doped SrTiO3 and BaTiO3 photocatalysts for hydrogen production from water without cocatalysts loading. International journal of hydrogen energy. 2012;37(22):17068-77.
CrossRef
- Yu H, Wang J, Yan S, Yu T, Zou Z. Elements doping to expand the light response of SrTiO3. Journal of Photochemistry and Photobiology A: Chemistry. 2014;275:65-71.
CrossRef
- Kang HW, Park SB. H2 evolution under visible light irradiation from aqueous methanol solution on SrTiO3: Cr/Ta prepared by spray pyrolysis from polymeric precursor. International journal of hydrogen energy. 2011;36(16):9496-504.
CrossRef
- Lu L, Ni S, Liu G, Xu X. Structural dependence of photocatalytic hydrogen production over La/Cr co-doped perovskite compound ATiO3 (A= Ca, Sr and Ba). International Journal of Hydrogen Energy. 2017;42(37):23539-47.
CrossRef
- Zhang H, Chen G, He X, Xu J. Electronic structure and photocatalytic properties of Ag–La codoped CaTiO3. Journal of alloys and compounds. 2012;516:91-5.
CrossRef
- Kang HW, Park SB. Improved performance of tri-doped photocatalyst SrTiO3: Rh/Ta/F for H2 evolution under visible light irradiation. International Journal of Hydrogen Energy. 2016;41(32):13970-8.
CrossRef
- Cao S, Low J, Yu J, Jaroniec M. Polymeric photocatalysts based on graphitic carbon nitride. Advanced Materials. 2015;27(13):2150-76.
CrossRef
- Liu J, Zhang T, Wang Z, Dawson G, Chen W. Simple pyrolysis of urea into graphitic carbon nitride with recyclable adsorption and photocatalytic activity. Journal of Materials Chemistry. 2011;21(38):14398-401.
CrossRef
- Pang X, Bian H, Wang W, Liu C, Khan MS, Wang Q, et al. A bio-chemical application of N-GQDs and g-C3N4 QDs sensitized TiO2 nanopillars for the quantitative detection of pcDNA3-HBV. Biosensors and Bioelectronics. 2017;91:456-64.
CrossRef
- Patnaik S, Martha S, Parida K. An overview of the structural, textural and morphological modulations of gC 3 N 4 towards photocatalytic hydrogen production. Rsc Advances. 2016;6(52):46929-51.
CrossRef
- Li C, Luo Z, Wang T, Gong J. Surface, Bulk, and Interface: Rational Design of Hematite Architecture toward Efficient Photo‐Electrochemical Water Splitting. Advanced Materials. 2018;30(30):1707502.
CrossRef
- Zhong Y, Wang Z, Feng J, Yan S, Zhang H, Li Z, et al. Improvement in photocatalytic H2 evolution over g-C3N4 prepared from protonated melamine. Applied surface science. 2014;295:253-9.
CrossRef
- Ma L, Fan H, Li M, Tian H, Fang J, Dong G. A simple melamine-assisted exfoliation of polymeric graphitic carbon nitrides for highly efficient hydrogen production from water under visible light. Journal of Materials Chemistry A. 2015;3(44):22404-12.
CrossRef
- Wang Y, Zhao S, Zhang Y, Fang J, Zhou Y, Yuan S, et al. One-pot synthesis of K-doped g-C3N4 nanosheets with enhanced photocatalytic hydrogen production under visible-light irradiation. Applied Surface Science. 2018;440:258-65.
CrossRef
- Liu M, Xia P, Zhang L, Cheng B, Yu J. Enhanced photocatalytic H2-production activity of g-C3N4 nanosheets via optimal photodeposition of Pt as cocatalyst. ACS Sustainable Chemistry & Engineering. 2018;6(8):10472-80.
CrossRef
- Maeda K, Domen K. Photocatalytic water splitting: recent progress and future challenges. The Journal of Physical Chemistry Letters. 2010;1(18):2655-61.
CrossRef
- Humayun M, Fu Q, Zheng Z, Li H, Luo W. Improved visible-light catalytic activities of novel Au/P-doped g-C3N4 photocatalyst for solar fuel production and mechanism. Applied Catalysis A: General. 2018;568:139-47.
CrossRef
- Yan X, Jia Z, Che H, Chen S, Hu P, Wang J, et al. A selective ion replacement strategy for the synthesis of copper doped carbon nitride nanotubes with improved photocatalytic hydrogen evolution. Applied Catalysis B: Environmental. 2018;234:19-25.
CrossRef
- Sun C, Zhang H, Liu H, Zheng X, Zou W, Dong L, et al. Enhanced activity of visible-light photocatalytic H2 evolution of sulfur-doped g-C3N4 photocatalyst via nanoparticle metal Ni as cocatalyst. Applied Catalysis B: Environmental. 2018;235:66-74.
CrossRef
- Qi K, Xie Y, Wang R, Liu S-y, Zhao Z. Electroless plating Ni-P cocatalyst decorated g-C3N4 with enhanced photocatalytic water splitting for H2 generation. Applied Surface Science. 2019;466:847-53.
CrossRef
- Chen P-W, Li K, Yu Y-X, Zhang W-D. Cobalt-doped graphitic carbon nitride photocatalysts with high activity for hydrogen evolution. Applied Surface Science. 2017;392:608-15.
CrossRef
- Jo W-K, Kumar S, Eslava S, Tonda S. Construction of Bi2WO6/RGO/g-C3N4 2D/2D/2D hybrid Z-scheme heterojunctions with large interfacial contact area for efficient charge separation and high-performance photoreduction of CO2 and H2O into solar fuels. Applied Catalysis B: Environmental. 2018;239:586-98.
CrossRef
- Huang Z, Li F, Chen B, Yuan G. Porous and low-defected graphitic carbon nitride nanotubes for efficient hydrogen evolution under visible light irradiation. RSC Advances. 2015;5(124):102700-6.
CrossRef
- Zhou Y, Zhang L, Huang W, Kong Q, Fan X, Wang M, et al. N-doped graphitic carbon-incorporated g-C3N4 for remarkably enhanced photocatalytic H2 evolution under visible light. Carbon. 2016;99:111-7.
CrossRef
- Chen J, Hong Z, Chen Y, Lin B, Gao B. One-step synthesis of sulfur-doped and nitrogen-deficient g-C3N4 photocatalyst for enhanced hydrogen evolution under visible light. Materials Letters. 2015;145:129-32.
CrossRef
- Lan Z-A, Zhang G, Wang X. A facile synthesis of Br-modified g-C3N4 semiconductors for photoredox water splitting. Applied Catalysis B: Environmental. 2016;192:116-25.
CrossRef
- Wang H, Bian Y, Hu J, Dai L. Highly crystalline sulfur-doped carbon nitride as photocatalyst for efficient visible-light hydrogen generation. Applied Catalysis B: Environmental. 2018;238:592-8.
CrossRef
- Wu M, Zhang J, He B-b, Wang H-w, Wang R, Gong Y-s. In-situ construction of coral-like porous P-doped g-C3N4 tubes with hybrid 1D/2D architecture and high efficient photocatalytic hydrogen evolution. Applied Catalysis B: Environmental. 2019;241:159-66.
CrossRef
- Zhang J-W, Gong S, Mahmood N, Pan L, Zhang X, Zou J-J. Oxygen-doped nanoporous carbon nitride via water-based homogeneous supramolecular assembly for photocatalytic hydrogen evolution. Applied Catalysis B: Environmental. 2018;221:9-16.
CrossRef
- Wang H, Yang C, Li M, Chen F, Cui Y. Enhanced photocatalytic hydrogen production of restructured B/F codoped g-C3N4 via post-thermal treatment. Materials Letters. 2018;212:319-22.
CrossRef
- Guo S, Tang Y, Xie Y, Tian C, Feng Q, Zhou W, et al. P-doped tubular g-C3N4 with surface carbon defects: universal synthesis and enhanced visible-light photocatalytic hydrogen production. Applied Catalysis B: Environmental. 2017;218:664-71.
CrossRef
- Zhang J, Huang F. Enhanced visible light photocatalytic H2 production activity of g-C3N4 via carbon fiber. Applied Surface Science. 2015;358:287-95.
CrossRef
- Wu Z, Gao H, Yan S, Zou Z. Synthesis of carbon black/carbon nitride intercalation compound composite for efficient hydrogen production. Dalton Transactions. 2014;43(31):12013-7.
CrossRef
- Li Z, Luo W, Zhang M, Feng J, Zou Z. Photoelectrochemical cells for solar hydrogen production: current state of promising photoelectrodes, methods to improve their properties, and outlook. Energy & Environmental Science. 2013;6(2):347-70.
CrossRef
- Park Y, McDonald KJ, Choi K-S. Progress in bismuth vanadate photoanodes for use in solar water oxidation. Chemical Society Reviews. 2013;42(6):2321-37.
CrossRef
- Jo WJ, Jang JW, Kong Kj, Kang HJ, Kim JY, Jun H, et al. Phosphate doping into monoclinic BiVO4 for enhanced photoelectrochemical water oxidation activity. Angewandte Chemie International Edition. 2012;51(13):3147-51.
CrossRef
- Jia Q, Iwashina K, Kudo A. Facile fabrication of an efficient BiVO4 thin film electrode for water splitting under visible light irradiation. Proceedings of the National Academy of Sciences. 2012;109(29):11564-9.
CrossRef
- Choi SK, Choi W, Park H. Solar water oxidation using nickel-borate coupled BiVO 4 photoelectrodes. Physical Chemistry Chemical Physics. 2013;15(17):6499-507.
CrossRef
- Lianos P. Review of recent trends in photoelectrocatalytic conversion of solar energy to electricity and hydrogen. Applied Catalysis B: Environmental. 2017;210:235-54.
CrossRef
- Fang L, Nan F, Yang Y, Cao D. Enhanced photoelectrochemical and photocatalytic activity in visible-light-driven Ag/BiVO4 inverse opals. Applied Physics Letters. 2016;108(9):093902.
CrossRef
- Zhong DK, Choi S, Gamelin DR. Near-complete suppression of surface recombination in solar photoelectrolysis by “Co-Pi” catalyst-modified W: BiVO4. Journal of the American Chemical Society. 2011;133(45):18370-7.
CrossRef
- Surendranath Y, Kanan MW, Nocera DG. Mechanistic studies of the oxygen evolution reaction by a cobalt-phosphate catalyst at neutral pH. Journal of the American Chemical Society. 2010;132(46):16501-9.
CrossRef
- Rohloff M, Anke B, Zhang S, Gernert U, Scheu C, Lerch M, et al. Mo-doped BiVO 4 thin films–high photoelectrochemical water splitting performance achieved by a tailored structure and morphology. Sustainable Energy & Fuels. 2017;1(8):1830-46.
CrossRef
- Soltani T, Tayyebi A, Lee B-K. Efficient promotion of charge separation with reduced graphene oxide (rGO) in BiVO4/rGO photoanode for greatly enhanced photoelectrochemical water splitting. Solar Energy Materials and Solar Cells. 2018;185:325-32.
CrossRef
- Wang T, Li C, Ji J, Wei Y, Zhang P, Wang S, et al. Reduced graphene oxide (rGO)/BiVO4 composites with maximized interfacial coupling for visible lght photocatalysis. ACS Sustainable Chemistry & Engineering. 2014;2(10):2253-8.
CrossRef
- Rohloff M, Anke Br, Kasian O, Zhang S, Lerch M, Scheu C, et al. Enhanced Photoelectrochemical Water Oxidation Performance by Fluorine Incorporation in BiVO4 and Mo: BiVO4 Thin Film Photoanodes. ACS applied materials & interfaces. 2019;11(18):16430-42.
CrossRef
- Kiani F, Astani NA, Rahighi R, Tayyebi A, Tayebi M, Khezri J, et al. Effect of graphene oxide nanosheets on visible light-assisted antibacterial activity of vertically-aligned copper oxide nanowire arrays. Journal of colloid and interface science. 2018;521:119-31.
CrossRef
- Luo W, Yang Z, Li Z, Zhang J, Liu J, Zhao Z, et al. Solar hydrogen generation from seawater with a modified BiVO4 photoanode. Energy & Environmental Science. 2011;4(10):4046-51.
CrossRef
- Yang Y, Kang L, Li H. Enhancement of photocatalytic hydrogen production of BiFeO3 by Gd3+ doping. Ceramics International. 2019;45(6):8017-22.
CrossRef
- Fujishima A, Honda K. Electrochemical evidence for the mechanism of the primary stage of photosynthesis. Bulletin of the chemical society of Japan. 1971;44(4):1148-50.
CrossRef
- Liu H, Xu C, Li D, Jiang HL. Photocatalytic hydrogen production coupled with selective benzylamine oxidation over MOF composites. Angewandte Chemie International Edition. 2018;57(19):5379-83.
CrossRef
- Goto Y, Hisatomi T, Wang Q, Higashi T, Ishikiriyama K, Maeda T, et al. A particulate photocatalyst water-splitting panel for large-scale solar hydrogen generation. Joule. 2018;2(3):509-20.
CrossRef
- Lyu H, Hisatomi T, Goto Y, Yoshida M, Higashi T, Katayama M, et al. An Al-doped SrTiO 3 photocatalyst maintaining sunlight-driven overall water splitting activity for over 1000 h of constant illumination. Chemical science. 2019;10(11):3196-201.
CrossRef
- Ohtani B. Preparing articles on photocatalysis—beyond the illusions, misconceptions, and speculation. Chemistry letters. 2008;37(3):216-29.
CrossRef
- Jeong H, Kim T, Kim D, Kim K. Hydrogen production by the photocatalytic overall water splitting on NiO/Sr3Ti2O7: effect of preparation method. International journal of hydrogen energy. 2006;31(9):1142-6.
CrossRef
- Nishimoto S, Matsuda M, Miyake M. Photocatalytic activities of Rh-doped CaTiO3 under visible light irradiation. Chemistry letters. 2006;35(3):308-9.
CrossRef
- Ko Y-G, Lee WY. Effects of nickel-loading method on the water-splitting activity of a layered NiO x/Sr 4 Ti 3 O 10 photocatalyst. Catalysis letters. 2002;83(3-4):157-60.
CrossRef
- Moon S-C, Mametsuka H, Suzuki E, Anpo M. Stoichiometric decomposition of pure water over Pt-loaded Ti/B binary oxide under UV-irradiation. Chemistry letters. 1998;27(2):117-8.
CrossRef
- Ikeda S, Hara M, Kondo JN, Domen K, Takahashi H, Okub T, et al. Preparation of a high active photocatalyst, K 2 La 2 Ti 3 O 10, by polymerized complex method and its photocatalytic activity of water splitting. Journal of materials research. 1998;13(4):852-5.
CrossRef
- Sayama K, Arakawa H. Effect of carbonate salt addition on the photocatalytic decomposition of liquid water over Pt–TiO 2 catalyst. Journal of the Chemical Society, Faraday Transactions. 1997;93(8):1647-54.
CrossRef
- Tabata S, Nishida H, Masaki Y, Tabata K. Stoichiometric photocatalytic decomposition of pure water in Pt/TiO 2 aqueous suspension system. Catalysis letters. 1995;34(1-2):245-9.
CrossRef
- Kudo A, Sayama K, Tanaka A, Asakura K, Domen K, Maruya K, et al. Nickel-loaded K4Nb6O17 photocatalyst in the decomposition of H2O into H2 and O2: Structure and reaction mechanism. Journal of catalysis. 1989;120(2):337-52.
- Yamaguti K, Sato S. Photolysis of water over metallized powdered titanium dioxide. Journal of the Chemical Society, Faraday Transactions 1: Physical Chemistry in Condensed Phases. 1985;81(5):1237-46.
- Lehn J. Photochemical water splitting continuous generation of hydrogen and oxygen by irradiation of aqueous suspensions of metal loaded strontium titanate. 1980.
- Domen K, Naito S, Soma M, Onishi T, Tamaru K. Photocatalytic decomposition of water vapour on an NiO–SrTiO 3 catalyst. Journal of the Chemical Society, Chemical Communications. 1980(12):543-4.
- Domen K, Naito S, Onishi T, Tamaru K, Soma M. Study of the photocatalytic decomposition of water vapor over a nickel (II) oxide-strontium titanate (SrTiO3) catalyst. The Journal of Physical Chemistry. 1982;86(18):3657-61.
- Lee WC, Tan L-L, Sumathi S, Chai S-P. Copper-doped flower-like molybdenum disulfide/bismuth sulfide photocatalysts for enhanced solar water splitting. International Journal of Hydrogen Energy. 2018;43(2):748-56.
- Wang SY, Ko TS, Huang CC, Huang YS. Optical and electrical properties of MoS2 and Fe-doped MoS2. Japanese Journal of Applied Physics. 2014;53(4S):04EH7.
- Lee WC, Gui M-M, Tan L-L, Wu TY, Sumathi S, Chai S-P. Bismuth sulphide-modified molybdenum disulphide as an efficient photocatalyst for hydrogen production under simulated solar light. Catalysis Communications. 2017;98:66-70.
- Li H, Tu W, Zhou Y, Zou Z. Z‐Scheme photocatalytic systems for promoting photocatalytic performance: recent progress and future challenges. Advanced Science. 2016;3(11):1500389.
- Yamaguti K, Sato S. Water photolysis over metallized SrTiO3 catalysts. Nouveau journal de chimie (1977). 1986;10(4-5):217-21.
- Domen K, Kudo A, Onishi T. Mechanism of photocatalytic decomposition of water into H2 and O2 over NiO SrTiO3. Journal of Catalysis. 1986;102(1):92-8.
- Domen K, Kudo A, Onishi T, Kosugi N, Kuroda H. Photocatalytic decomposition of water into hydrogen and oxygen over nickel (II) oxide-strontium titanate (SrTiO3) powder. 1. Structure of the catalysts. The Journal of Physical Chemistry. 1986;90(2):292-5.
- Miseki Y, Kato H, Kudo A. Water splitting into H2 and O2 over Cs2Nb4O11 photocatalyst. Chemistry letters. 2005;34(1):54-5.
- Ebina Y, Sakai N, Sasaki T. Photocatalyst of lamellar aggregates of RuO x-loaded perovskite nanosheets for overall water splitting. The Journal of Physical Chemistry B. 2005;109(36):17212-6.
- Kudo A, Tanaka A, Domen K, Onishi T. The effects of the calcination temperature of SrTiO3 powder on photocatalytic activities. Journal of Catalysis. 1988;111(2):296-301.
- Abe R, Higashi M, Sayama K, Abe Y, Sugihara H. Photocatalytic activity of R3MO7 and R2Ti2O7 (R= Y, Gd, La; M= Nb, Ta) for water splitting into H2 and O2. The Journal of Physical Chemistry B. 2006;110(5):2219-26.
- Abe R, Higashi M, Zou Z, Sayama K, Abe Y. Photocatalytic water splitting into H2 and O2 over R2Ti2O7 (R= Y, rare earth) with pyrochlore structure. Chemistry letters. 2004;33(8):954-5.
- Higashi M, Abe R, Sayama K, Sugihara H, Abe Y. Improvement of photocatalytic activity of titanate pyrochlore Y2Ti2O7 by addition of excess Y. Chemistry letters. 2005;34(8):1122-3.
- Reddy VR, Hwang DW, Lee JS. Effect of Zr substitution for Ti in KLaTiO 4 for photocatalytic water splitting. Catalysis letters. 2003;90(1-2):39-43.
- Kudo A, Okutomi H, Kato H. Photocatalytic water splitting into H2 and O2 over K2LnTa5O15 powder. Chemistry letters. 2000;29(10):1212-3.
- Takata T, Furumi Y, Shinohara K, Tanaka A, Hara M, Kondo JN, et al. Photocatalytic decomposition of water on spontaneously hydrated layered perovskites. Chemistry of materials. 1997;9(5):1063-4.
- Sreethawong T, Suzuki Y, Yoshikawa S, editors. Improved photocatalytic hydrogen evolution over mesoporous Ni/TiO2 prepared by single-step sol-gel technique with surfactant template. Electron Transfer in Nanomaterials: Proceedings of the International Symposium; 2006: The Electrochemical Society.
- Kato H, Kudo A. New tantalate photocatalysts for water decomposition into H2 and O2. Chemical Physics Letters. 1998;295(5-6):487-92.
- Hong SJ, Jun H, Lee JS. Nanocrystalline WO3 film with high photo-electrochemical activity prepared by polymer-assisted direct deposition. Scripta Materialia. 2010;63(7):757-60.
- Chatchai P, Murakami Y, Kishioka S-y, Nosaka AY, Nosaka Y. Efficient photocatalytic activity of water oxidation over WO3/BiVO4 composite under visible light irradiation. Electrochimica Acta. 2009;54(3):1147-52.
- Chatchai P, Kishioka S-y, Murakami Y, Nosaka AY, Nosaka Y. Enhanced photoelectrocatalytic activity of FTO/WO3/BiVO4 electrode modified with gold nanoparticles for water oxidation under visible light irradiation. Electrochimica acta. 2010;55(3):592-6.
- Chatchai P, Murakami Y, Kishioka S-y, Nosaka A, Nosaka Y. FTO∕ SnO2∕ BiVO4 composite photoelectrode for water oxidation under visible light irradiation. Electrochemical and Solid-State Letters. 2008;11(6):H160-H3.
- Byun S, Kim B, Jeon S, Shin B. Effects of a SnO 2 hole blocking layer in a BiVO 4-based photoanode on photoelectrocatalytic water oxidation. Journal of Materials Chemistry A. 2017;5(15):6905-13.
- Xia L, Bai J, Li J, Zeng Q, Li L, Zhou B. High-performance BiVO4 photoanodes cocatalyzed with an ultrathin α-Fe2O3 layer for photoelectrochemical application. Applied Catalysis B: Environmental. 2017;204:127-33.
- Kim JH, Jang J-W, Jo YH, Abdi FF, Lee YH, Van De Krol R, et al. Hetero-type dual photoanodes for unbiased solar water splitting with extended light harvesting. Nature communications. 2016;7(1):1-9.
- Pilli SK, Deutsch TG, Furtak TE, Brown LD, Turner JA, Herring AM. BiVO 4/CuWO 4 heterojunction photoanodes for efficient solar driven water oxidation. Physical chemistry chemical physics. 2013;15(9):3273-8.
- Li L-P, Liu M, Zhang W-D. Electrodeposition of CdS onto BiVO 4 films with high photoelectrochemical performance. Journal of Solid State Electrochemistry. 2018;22(8):2569-77.
- Jiang J, Wang M, Li R, Ma L, Guo L. Fabricating CdS/BiVO4 and BiVO4/CdS heterostructured film photoelectrodes for photoelectrochemical applications. International journal of hydrogen energy. 2013;38(29):13069-76.
- Su J, Guo L, Bao N, Grimes CA. Nanostructured WO3/BiVO4 heterojunction films for efficient photoelectrochemical water splitting. Nano letters. 2011;11(5):1928-33.
- Moniz SJ, Zhu J, Tang J. 1D Co‐Pi modified BiVO4/ZnO junction cascade for efficient photoelectrochemical water cleavage. Advanced Energy Materials. 2014;4(10):1301590.
- Ho-Kimura S, Moniz SJ, Handoko AD, Tang J. Enhanced photoelectrochemical water splitting by nanostructured BiVO 4–TiO 2 composite electrodes. Journal of Materials Chemistry A. 2014;2(11):3948-53.
- Gurunathan K, Maruthamuthu P, Sastri M. Photocatalytic hydrogen production by dye-sensitized Pt/SnO2 and Pt/SnO2/RuO2 in aqueous methyl viologen solution. International Journal of Hydrogen Energy. 1997;22(1):57-62.
- Dhanalakshmi K, Latha S, Anandan S, Maruthamuthu P. Dye sensitized hydrogen evolution from water. International Journal of Hydrogen Energy. 2001;26(7):669-74.
- Polo AS, Itokazu MK, Iha NYM. Metal complex sensitizers in dye-sensitized solar cells. Coordination Chemistry Reviews. 2004;248(13-14):1343-61.
- Bi Z-C, Tien HT. Photoproduction of hydrogen by dye-sensitized systems. International Journal of Hydrogen Energy. 1984;9(8):717-22.
- Ni M, Leung MK, Leung DY, Sumathy K. A review and recent developments in photocatalytic water-splitting using TiO2 for hydrogen production. Renewable and Sustainable Energy Reviews. 2007;11(3):401-25.
- Gole JL, Stout JD, Burda C, Lou Y, Chen X. Highly efficient formation of visible light tunable TiO2-x N x photocatalysts and their transformation at the nanoscale. The journal of physical chemistry B. 2004;108(4):1230-40.
- Mrowetz M, Balcerski W, Colussi A, Hoffmann MR. Oxidative power of nitrogen-doped TiO2 photocatalysts under visible illumination. The Journal of Physical Chemistry B. 2004;108(45):17269-73.
- Wu N-L, Lee M-S. Enhanced TiO2 photocatalysis by Cu in hydrogen production from aqueous methanol solution. International Journal of Hydrogen Energy. 2004;29(15):1601-5.
- Szabó-Bárdos E, Czili H, Horváth A. Photocatalytic oxidation of oxalic acid enhanced by silver deposition on a TiO2 surface. Journal of Photochemistry and Photobiology A: Chemistry. 2003;154(2-3):195-201.
- Anpo M, Takeuchi M. The design and development of highly reactive titanium oxide photocatalysts operating under visible light irradiation. Journal of catalysis. 2003;216(1-2):505-16.
- Subramanian V, Wolf EE, Kamat PV. Catalysis with TiO2/gold nanocomposites. Effect of metal particle size on the Fermi level equilibration. Journal of the American Chemical Society. 2004;126(15):4943-50.
- Subramanian V, Wolf EE, Kamat PV. Green emission to probe photoinduced charging events in ZnO− Au nanoparticles. Charge distribution and Fermi-level equilibration. The Journal of Physical Chemistry B. 2003;107(30):7479-85.
- Jakob M, Levanon H, Kamat PV. Charge distribution between UV-irradiated TiO2 and gold nanoparticles: determination of shift in the Fermi level. Nano letters. 2003;3(3):353-8.
- Bamwenda GR, Tsubota S, Nakamura T, Haruta M. Photoassisted hydrogen production from a water-ethanol solution: a comparison of activities of Au TiO2 and Pt TiO2. Journal of Photochemistry and Photobiology A: Chemistry. 1995;89(2):177-89.
- Sakthivel S, Shankar M, Palanichamy M, Arabindoo B, Bahnemann D, Murugesan V. Enhancement of photocatalytic activity by metal deposition: characterisation and photonic efficiency of Pt, Au and Pd deposited on TiO2 catalyst. Water research. 2004;38(13):3001-8.
- Balandin AA, Ghosh S, Bao W, Calizo I, Teweldebrhan D, Miao F, et al. Superior thermal conductivity of single-layer graphene. Nano letters. 2008;8(3):902-7.
- Martin J, Akerman N, Ulbricht G, Lohmann T, Smet Jv, Von Klitzing K, et al. Observation of electron–hole puddles in graphene using a scanning single-electron transistor. Nature physics. 2008;4(2):144-8.
- Zhang X-Y, Li H-P, Cui X-L, Lin Y. Graphene/TiO 2 nanocomposites: synthesis, characterization and application in hydrogen evolution from water photocatalytic splitting. Journal of Materials Chemistry. 2010;20(14):2801-6.
- Xie H, Hou C, Wang H, Zhang Q, Li Y. S, N co-doped graphene quantum dot/TiO 2 composites for efficient photocatalytic hydrogen generation. Nanoscale research letters. 2017;12(1):400.
- Fan W, Lai Q, Zhang Q, Wang Y. Nanocomposites of TiO2 and reduced graphene oxide as efficient photocatalysts for hydrogen evolution. The Journal of Physical Chemistry C. 2011;115(21):10694-701.
- Hao X, Jin Z, Xu J, Min S, Lu G. Functionalization of TiO2 with graphene quantum dots for efficient photocatalytic hydrogen evolution. Superlattices and Microstructures. 2016;94:237-44.
- Zhang K, Jing D, Xing C, Guo L. Significantly improved photocatalytic hydrogen production activity over Cd1-xZnxS photocatalysts prepared by a novel thermal sulfuration method. International Journal of Hydrogen Energy. 2007;32(18):4685-91.
- Zahraa MBaO. Photocatalytic reactors. INTERNATIONAL JOURNAL OF PHOTOENERGY. 2003;05.
- Hisatomi T, Domen K. Reaction systems for solar hydrogen production via water splitting with particulate semiconductor photocatalysts. Nature Catalysis. 2019;2(5):387-99.
- Nguyen T-V, Wu JC. Photoreduction of CO2 to fuels under sunlight using optical-fiber reactor. Solar energy materials and solar cells. 2008;92(8):864-72.
- Ola O, Maroto-Valer MM. Review of material design and reactor engineering on TiO2 photocatalysis for CO2 reduction. Journal of Photochemistry and Photobiology C: Photochemistry Reviews. 2015;24:16-42.
- Hu Y, Xu J, Yuan C, Lin J, Yin Z. A single TiO 2-coated side-glowing optical fiber for photocatalytic wastewater treatment. Chinese Science Bulletin. 2005;50(18):1979-84.
- Tahir M, Tahir B, Amin NS. Photocatalytic CO2 reduction by CH4 over montmorillonite modified TiO2 nanocomposites in a continuous monolith photoreactor. Materials Research Bulletin. 2015;63:13-23.
- Boyjoo Y, Sun H, Liu J, Pareek VK, Wang S. A review on photocatalysis for air treatment: from catalyst development to reactor design. Chemical Engineering Journal. 2017;310:537-59.
- Gaudillere C, González JJ, Chica A, Serra JM. YSZ monoliths promoted with Co as catalysts for the production of H2 by steam reforming of ethanol. Applied Catalysis A: General. 2017;538:165-73.
- Tahir M, Amin NS. Photocatalytic CO2 reduction and kinetic study over In/TiO2 nanoparticles supported microchannel monolith photoreactor. Applied Catalysis A: General. 2013;467:483-96.
- Tahir M, Amin NS. Performance analysis of nanostructured NiO–In2O3/TiO2 catalyst for CO2 photoreduction with H2 in a monolith photoreactor. Chemical Engineering Journal. 2016;285:635-49.
- Pinaud BA, Benck JD, Seitz LC, Forman AJ, Chen Z, Deutsch TG, et al. Technical and economic feasibility of centralized facilities for solar hydrogen production via photocatalysis and photoelectrochemistry. Energy & Environmental Science. 2013;6(7):1983-2002.
- Setoyama T, Takewaki T, Domen K, Tatsumi T. The challenges of solar hydrogen in chemical industry: how to provide, and how to apply? Faraday discussions. 2017;198:509-27.
- Jing D, Liu H, Zhang X, Zhao L, Guo L. Photocatalytic hydrogen production under direct solar light in a CPC based solar reactor: reactor design and preliminary results. Energy Conversion and Management. 2009;50(12):2919-26.
- Tahir M, Amin NS. Recycling of carbon dioxide to renewable fuels by photocatalysis: Prospects and challenges. Renewable and Sustainable Energy Reviews. 2013;25:560-79.
- Lin H, Valsaraj KT. An optical fiber monolith reactor for photocatalytic wastewater treatment. AIChE journal. 2006;52(6):2271-80.
- Al-Hamdi AM, Rinner U, Sillanpää M. Tin dioxide as a photocatalyst for water treatment: a review. Process Safety and Environmental Protection. 2017;107:190-205.
- Puga AV. Photocatalytic production of hydrogen from biomass-derived feedstocks. Coordination Chemistry Reviews. 2016;315:1-66.
- Ahmad H, Kamarudin S, Minggu L, Kassim M. Hydrogen from photo-catalytic water splitting process: A review. Renewable and Sustainable Energy Reviews. 2015;43:599-610.
- Baniasadi E, Dincer I, Naterer G. Preformance analysis of a water splitting reactor with hybrid photochemical conversion of solar energy. International journal of hydrogen energy. 2012;37(9):7464-72.
- Tambago HMG, de Leon RL. Intrinsic kinetic modeling of hydrogen production by photocatalytic water splitting using cadmium zinc sulfide catalyst. Int J Chem Eng Appl. 2015;6:220-7.
- Kumar DP, Kumari VD, Karthik M, Sathish M, Shankar M. Shape dependence structural, optical and photocatalytic properties of TiO2 nanocrystals for enhanced hydrogen production via glycerol reforming. Solar Energy Materials and Solar Cells. 2017;163:113-9.
- Boudjemaa A, Rebahi A, Terfassa B, Chebout R, Mokrani T, Bachari K, et al. Fe2O3/carbon spheres for efficient photo-catalytic hydrogen production from water and under visible light irradiation. Solar Energy Materials and Solar Cells. 2015;140:405-11.
- Huaxu L, Fuqiang W, Ziming C, Shengpeng H, Bing X, Xiangtao G, et al. Analyzing the effects of reaction temperature on photo-thermo chemical synergetic catalytic water splitting under full-spectrum solar irradiation: an experimental and thermodynamic investigation. International Journal of Hydrogen Energy. 2017;42(17):12133-42.
- Zhang Z, Maggard PA. Investigation of photocatalytically-active hydrated forms of amorphous titania, TiO2· nH2O. Journal of Photochemistry and Photobiology A: Chemistry. 2007;186(1):8-13.
- Liu S, Luo Z, Li L, Li H, Chen M, Wang T, et al. Multifunctional TiO2 overlayer for p-Si/n-CdS heterojunction photocathode with improved efficiency and stability. Nano Energy. 2018;53:125-9.
- Brahimi R, Bessekhouad Y, Bouguelia A, Trari M. CuAlO2/TiO2 heterojunction applied to visible light H2 production. Journal of Photochemistry and Photobiology A: Chemistry. 2007;186(2-3):242-7.
- Maeda K. Photocatalytic properties of rutile TiO 2 powder for overall water splitting. Catalysis Science & Technology. 2014;4(7):1949-53.
- Fujita S-i, Kawamori H, Honda D, Yoshida H, Arai M. Photocatalytic hydrogen production from aqueous glycerol solution using NiO/TiO2 catalysts: Effects of preparation and reaction conditions. Applied Catalysis B: Environmental. 2016;181:818-24.
- Nada A, Hamed H, Barakat M, Mohamed N, Veziroglu T. Enhancement of photocatalytic hydrogen production rate using photosensitized TiO2/RuO2-MV2+. International journal of hydrogen energy. 2008;33(13):3264-9.
- Lu J, Wang Y, Huang J, Fei J, Cao L, Li C. In situ synthesis of mesoporous C-doped TiO2 single crystal with oxygen vacancy and its enhanced sunlight photocatalytic properties. Dyes and Pigments. 2017;144:203-11.
- Zhang D, Ma X, Zhang H, Liao Y, Xiang Q. Enhanced photocatalytic hydrogen evolution activity of carbon and nitrogen self-doped TiO2 hollow sphere with the creation of oxygen vacancy and Ti3+. Materials today energy. 2018;10:132-40.
- Méndez JO, López CR, Melián EP, Díaz OG, Rodríguez JD, Hevia DF, et al. Production of hydrogen by water photo-splitting over commercial and synthesised Au/TiO2 catalysts. Applied Catalysis B: Environmental. 2014;147:439-52.
- Chen W-T, Chan A, Al-Azri ZH, Dosado AG, Nadeem MA, Sun-Waterhouse D, et al. Effect of TiO2 polymorph and alcohol sacrificial agent on the activity of Au/TiO2 photocatalysts for H2 production in alcohol–water mixtures. Journal of catalysis. 2015;329:499-513.
- Police AKR, Basavaraju S, Valluri DK, Machiraju S, Lee JS. CaFe2O4 sensitized hierarchical TiO2 photo composite for hydrogen production under solar light irradiation. Chemical engineering journal. 2014;247:152-60.
- Umer M, Tahir M, Azam MU, Jaffar MM. Metals free MWCNTs@ TiO2@ MMT heterojunction composite with MMT as a mediator for fast charges separation towards visible light driven photocatalytic hydrogen evolution. Applied Surface Science. 2019;463:747-57.
- López-Tenllado F, Hidalgo-Carrillo J, Montes V, Marinas A, Urbano F, Marinas J, et al. A comparative study of hydrogen photocatalytic production from glycerol and propan-2-ol on M/TiO2 systems (M= Au, Pt, Pd). Catalysis Today. 2017;280:58-64.
- Cao B, Li G, Li H. Hollow spherical RuO2@ TiO2@ Pt bifunctional photocatalyst for coupled H2 production and pollutant degradation. Applied Catalysis B: Environmental. 2016;194:42-9.
- Al-Azri ZH, Chen W-T, Chan A, Jovic V, Ina T, Idriss H, et al. The roles of metal co-catalysts and reaction media in photocatalytic hydrogen production: Performance evaluation of M/TiO2 photocatalysts (M= Pd, Pt, Au) in different alcohol–water mixtures. Journal of catalysis. 2015;329:355-67.
- Li Y, Wang B, Liu S, Duan X, Hu Z. Synthesis and characterization of Cu2O/TiO2 photocatalysts for H2 evolution from aqueous solution with different scavengers. Applied Surface Science. 2015;324:736-44.
Views: 1,134
This work is licensed under a Creative Commons Attribution 4.0 International License.